The views expressed in this paper are those of the writer(s) and are not necessarily those of the ARJ Editor or Answers in Genesis.
Abstract
The Bible’s Flood account describes fountains bursting forth followed by enormous rain, global sea level rise, the death and the blotting out of air breathing land animals, along with man. The following model is proposed:
1. The initial Flood stage was characterized by venting of acidic water and rainfall, together with high energy erosion and deposition. Upper Proterozoic mixtites1 formed from mass flows downslope from land into the seas.
2. The blotting out process meant the death of terrestrial vertebrates (land animals) everywhere, as well as the dissolution of vertebrate bones (and invertebrate shells) under acidic and erosive conditions in the vicinity of volcanic fountains.
3. On shelfal areas at greater distances from the fountains, ocean chemistry was more normal, that is, slightly alkaline. This enabled dissolved bones (and shells) to precipitate out as amorphous phosphorite deposits. All continents excepting Antarctica, to date, are known to have Precambrian-Cambrian transition phosphorites and these phosphorite deposits are generally found stratigraphically above Upper Proterozoic mixtites.
Thus this paper highlights geochemical evidence within a biblical Flood framework and proposes that terrestrial vertebrates close to the Flood fountains were dissolved and then precipitated out to form Precambrian-Cambrian transition sedimentary phosphate deposits.
Introduction
Although the radiometric date for the base of the Cambrian has been revised by secular scientists, the relative position of Precambrian and Cambrian strata remains observable and mappable, and recognized by geologists (including a number of young-earth creationists) worldwide. Cambrian strata are recognized by the lowermost appearance of shelly fossils (of nearly every major invertebrate animal group).
Rocks of the Precambrian-Cambrian transition point to one of the greatest upheavals in the earth’s history. This transition is widely regarded by geologists as of great importance in terms of phosphogenesis, ocean chemistry, tectonism, volcanism, erosion, deposition, sea level rise, and the fossil record (Brasier 1992; Cook and Shergold 1984; Halverson et al. 2009; Knoll and Walter 1992; McKenzie et al. 2014; Peters and Gaines 2012; Tucker 1992). The authors believe that the strata of the Precambrian-Cambrian transition and their chemical signature have immense significance when interpreted in terms of the onset of the Noahic Flood.
This paper elaborates on a paragraph in an earlier paper (Dickens and Snelling 2008):
In the vicinity of active acidic volcanic fountains the abrasive power of jets of water with entrained sediment assisted the solution of vertebrate bones and flesh. Abrasion would have provided finer grained material which would have assisted the chemical solution process. The dissolved material may have subsequently precipitated out as massive phosphorite deposits at localities around the Precambrian-Cambrian sequence boundary.
The focus of this paper is the relationship between the Flood fountains, mass flows (represented by Upper Proterozoic mixtites) and phosphorites which are located stratigraphically above the Upper Proterozoic mixtites. Scientists with an old age worldview do not recognize the connection between Proterozoic rock-hosted ore deposits, Upper Proterozoic mixtites, and Cambrian phosphorites found in locations such as northwest Queensland. This is because their deep time perspective entails the belief that there are many millions of years of time gaps between the formation of each of these three sets of geological deposits.
Geochemistry has the potential to greatly refine and enhance young-earth creation models of geology and the Bible, and in the case of the paper under review, to model effects early in Noah’s Flood.
Event Sequence
Cataclysmic judgment foretold
And God said to Noah, “I have determined to make an end of all flesh, for the earth is filled with violence through them. Behold, I will destroy them with the earth. (Genesis 6:13 ESV)
So the Lord said, “I will blot out man whom I have created from the face of the land, man and animals and creeping things and birds of the heavens, for I am sorry that I have made them.” (Genesis 6:7 ESV)
For behold, I will bring a flood of waters upon the earth to destroy all flesh in which is the breath of life under heaven. Everything that is on the earth shall die. (Genesis 6:17 ESV)
The breath of life implies the inclusion of land vertebrates (amphibians, reptiles, birds, and mammals including man). These animals breathe with lungs supported by a backbone, and bone is a unique characteristic of the skeletons of vertebrates (Pough, Heiser, and McFarland 1989, 57). Phosphorite (marine sedimentary phosphate) and vertebrate bones have essentially the same composition—calcium phosphate (Cook and Shergold 2005; Martin 2007).
Land animals and man in the vicinity of the Flood fountains were not to be annihilated as in the sense of going to nothing. Instead their life and structure was to be destroyed. “Destroy them with the earth” meant that the crust of the earth itself also was to be transformed but not reduced to nothing by way of the Flood.
Flood Tectonism
In the six hundredth year of Noah’s life, in the second month, on the seventeenth day of the month, on that day all the fountains of the great deep burst forth, and the windows of the heavens were opened. (Genesis 7:11 ESV)
by His knowledge the deeps broke open, . . . (Proverbs 3:20a ESV)
On a specific day there was simultaneous worldwide fracturing of earth’s crust as the fountains burst forth. “Fountains of the great deep burst forth” and “deeps broke open” imply rifting and fracturing of the earth’s crust. The text implies that water flowed from within the earth through the fountains, and rain fell. Volcanic material would have accompanied these eruptions as the crust ruptured, releasing pressure from the rocks underneath (Schmandt et al. 2014). Much of the water for the Noahic Flood likely came from various depths within the earth, with the mantle being the major water source (Bergeron 1997; Pearson et al. 2014; Schmandt et al 2014).
The Precambrian-Cambrian transition was characterized by rifting and continental extension (Ilyin 1990). Rifting and basin development is associated with supercontinent breakup. Reconstruction of the Rodinia supercontinent was based on recognition of correlatable Upper Proterozoic rifted passive margins (Evans 2013). Plume-related extensional events are indicated by reconstructions of voluminous mafic magmatism along the margins of paleo-continents where numerous mafic dyke swarms were inferred to form as hot rock rose through the earth during continental rifting (Pisarevsky et al. 2008).
A model of catastrophic plate tectonics during the global Flood has been proposed as follows (Austin et al. 1994). Flooding of the continents began with meters per second subduction of slabs of pre-Flood mafic oceanic floor along thousands of kilometers of pre-Flood continental margins. Flow induced in the mantle would have produced rapid extension along linear belts in the seafloor and corresponding rapid horizontal movement of the broken-apart continents. Upwelling magma jettisoned steam into the atmosphere causing intense global rain.
Most of the volume of modern volcanic gases is water in the form of water vapor (steam) as well as carbon dioxide. Other main volcanic gases include sulphur dioxide, hydrogen chloride, hydrogen sulphide, and hydrogen fluoride (Macdonald 1972; Sigurdsson et al. 2015). Volcanic gas aerosols include sulfuric acid and hydrochloric acid droplets with absorbed halide salts and so volcanic gas emissions are acidic and may contribute to acid rain (McGee et al. 1997) (see fig. 1).
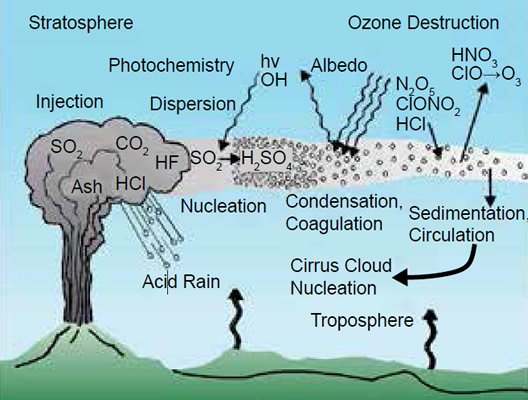
Fig. 1. Schematic of chemical effects from volcano injection of aerosols and gases into the atmosphere (after McGee et al. 1997).
Plate tectonic activity is inferred to have raised the level of carbon dioxide in the atmosphere and seawater through increased volcanism and subduction zone metamorphism. Tectonic outgassing is a major source of carbon dioxide and rapid carbon dioxide fluxes from large igneous province volcanism can cause dramatic environmental perturbations. Major pulses of volcanism and magmatism have been indicated by Cambrian strata based on zircon “age” data (McKenzie et al. 2014). The Cambrian had the highest modelled atmospheric carbon dioxide concentrations of the Phanerozoic (Berner 1990, 2006) (see fig. 2), “suggesting an extraordinary event at that time” (Cook 1992). This peak in partial pressure of atmospheric carbon dioxide may correspond with maximum pressure release and maximum output of carbon dioxide associated with the fountains of the great deep breaking forth early in Noah’s Flood.
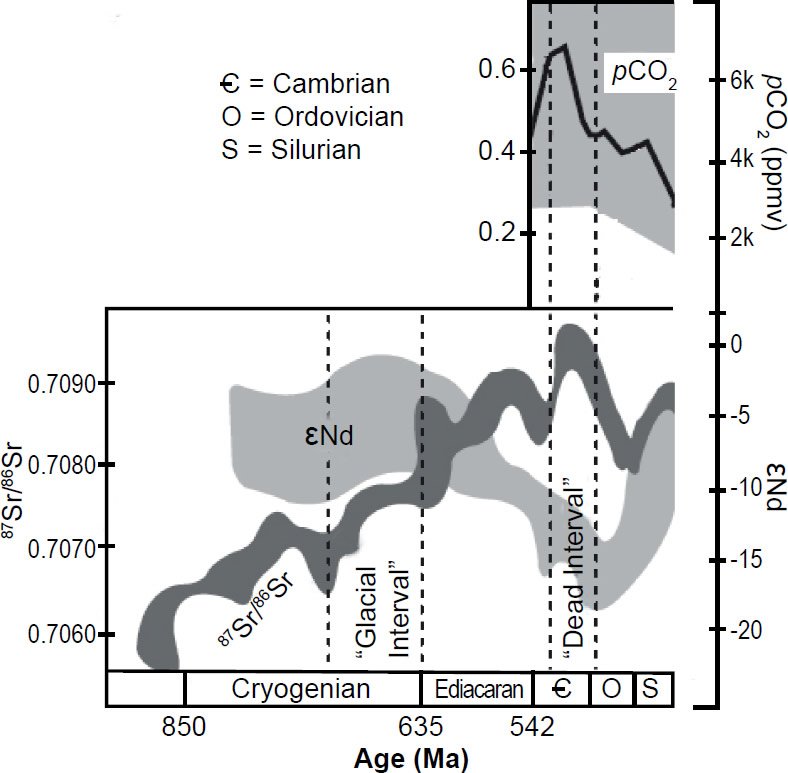
Fig. 2. Upper Proterozoic to Lower Paleozoic seawater strontium and neodymium isotopic trends, and partial pressure of atmospheric carbon dioxide (after McKenzie et al. 2014).
Detrital zircon “age” data indicate spatially extensive Ediacaran-Cambrian continental arc volcanism. Zircon is a common accessory mineral in felsic-intermediate igneous rocks and convergent margins with continental arcs produce voluminous felsic-intermediate magmas with high zircon abundance. The marked increase in “end-Cryogenian” detrital zircon in global distributions supports a major increase in continental arc volcanism at the “end of the “glacial”” interval (McKenzie et al. 2014).
Chemical effects decrease with increasing distance from modern erupting volcanoes (McGee et al. 1997). Thus, in Noah’s Flood, we believe that marine life would likely have been able to survive in higher proportions at increasing distances away from the fountains of the great deep.
A number of Precambrian-Cambrian transition black shales have been found to contain highly anomalous metal contents (Cook 1992; Donnelly, Shergold, and Southgate 1988; Wille et al. 2008; Xu et al. 2011). This includes highly metalliferous black shales over inferred carbonate shelves of south China, India, and Arabia (Brasier 1992). These metals are consistent with volcanic/fumarolic input into the system (Cook 1992). In addition, most metal deposits hosted in Proterozoic sedimentary and volcanic rocks can be shown to have formed in tectonic environments characterized by rifting (Sawkins 1983). Thus input of metals into Precambrian-Cambrian transition rocks and Proterozoic host rocks is believed to have occurred during the initial rifting and volcanism early in the Flood.
Continental Erosion and Mass Flows
And rain fell upon the earth forty days and forty nights. (Genesis 7:12 ESV)
and they were unaware until the flood came and swept them all away, so will be the coming of the Son of Man. (Matthew 24:39 ESV)
This rain event was the greatest rain event ever recorded. Erosion was consequently immense. The tremendous runoff from continents during the early stage of the Flood would have been responsible for the drawdown of carbon dioxide described for the Cryogenian (Berner 2006), as chemical weathering of silicate rocks is a major carbon dioxide sink (Berner, Lasaga, and Garrels 1983; Kump, Brantley, and Arthur 2000).
The rise in Upper Proterozoic strontium isotope ratios 87Sr/86Sr (see fig. 2) has been explained by accelerated rates of erosion associated with uplift during the so-called Pan-African orogeny (Derry et al. 1994). 87Sr/86Sr ratios (measured from shells) in Cambrian sedimentary carbonates are the highest of Phanerozoic strata (Veizer and Compston 1974; Veizer et al. 1999) (see fig. 2) and high crustal erosion rates have been inferred from Cambrian 87Sr/86Sr values (Derry et al. 1994). The large supplies of clastic sediments that flooded into basins imply high rates of erosion of the basin hinterlands, which in turn may explain the progressive rise in 87Sr/86Sr, as well as the very negative neodymium isotope ratio εNd(t) in the Cambrian2 (see fig. 2). Rubidium (Rb) is common in the crust’s silicate minerals (Faure and Powell 1972). 87Sr is a radiogenic daughter isotope of 87Rb. Therefore the abundance of radiogenic 87Sr relative to “common” 86Sr in a sample of sediment is related to the amount of sediment that originated from continental crust as opposed to that originating from the ocean. Similarly, a negative εNd(t) is related to fractionation of neodymium isotopes observed in crustal rocks compared to a standard.
Skeletal fragments in some Cambrian sediments have been described as attesting to abrasion by current activity (Donnelly, Shergold, and Southgate 1988). Abrasion of organisms with phosphatic hard parts (such as trilobites and brachiopods) to form pelletal phosphorites has also been described (Cook and McElhinny 1979).
Along with massive erosion of the continental landmasses, enormous torrential rain would have caused huge mass flows sweeping down from landmasses into the seas, depositing immense volumes of sediments. Upper Proterozoic mixtites of the North American Cordillera have been interpreted as submarine mass flow deposits (Austin and Wise 1994; Sigler and Wingerden 1998; Snelling 2009; Wingerden 2003) formed in the initial phase of the Flood rather than as glacial deposits. Upper Proterozoic mixtites are widespread, and are found in other regions, such as the Appalachian Mountains, Caledonian Mountains, Scandinavia, Russian Platform, Siberia, northwest China, Brazil, central and south Africa, and northwest, central and southern Australia (Schermerhorn 1974).
The Cryogenian was so named in mainstream scientific literature because abundant mixtites were interpreted as being formed in a cold glacial environment. The “snowball earth” concept has even been put forward based on interpreted equatorial latitudes for claimed Upper Proterozoic “glacial” rock units and on data indicating collapsed biological productivity (Arnaud, Halverson, and Shields-Zhou 2011; Hoffman et al. 1998; Shields 2005). However, Upper Proterozoic mixtites more likely represent mass flows down slopes adjacent to active tectonic areas since they occur by preference in thick mobile belt successions or unstable platforms, and not in the thin sequences developed in stable environments (Schermerhorn 1974). These mixtites formed along active tectonic zones (Austin and Wise 1994; Sigler and Wingerden 1998; Young 1995). Under a mass flow hypothesis, tectonically elevated borderlands would have provided freshly eroded material and the gradient necessary to transport it into adjacent basins. The mass flows in this environment would commence as subaerial flows, until entering the sea where they spread out as coastal fans, depositing their loads on loss of momentum (Schermerhorn 1974). Classic Upper Proterozoic “tillites” may be better interpreted as mass flow deposits (Oard 1997).
The occurrence of carbonate beds above, below, and within mixtite beds sets Upper Proterozoic mixtite formations apart from the claimed Middle Precambrian and Permo-Carboniferous glacial formations, and the genuine Pleistocene glacial formations (Schermerhorn 1974). The abundance of Proterozoic carbonates with stromatolites and ooids suggest a relatively warm rather than a cold climate prevailed at the time of deposition (Hallam 1981). Flat-pebble breccias also suggest warm depositional conditions, as they are formed in modern environments by the erosional rip-up of finely layered sedimentary rock (Brasier 1992; Roehl and Choquette 1985).
Transfer of atmospheric carbon dioxide to the ocean has been interpreted as enabling rapid precipitation of calcium carbonate in warm surface waters, producing the cap carbonate rocks over Upper Proterozoic mixtites which are observed globally (Shields 2005). It has been proposed that cap carbonates formed primarily by microbially mediated precipitation during algal blooms under low salinity conditions (Shields 2005). In addition, the presence of a very distinctive fine-grained carbonate facies (flakestone) provides evidence for extensive calcium carbonate precipitation (Tucker 1992). Carbon dioxide build-up from active Flood fountains is believed to have contributed to this rapid precipitation of calcium carbonate.
Structures interpreted as giant wave ripples (generated by sea surface waves) have been observed in cap carbonate rocks from Australia, Brazil, Canada, Namibia, and Svalbard (Allen and Hoffman 2005). A flakestone facies, with its characteristic broken-up clasts, is a further indicator of the action of stormy seas (Tucker 1992). Waves would not have been possible in an ice-covered ocean. Instead giant tsunami waves would have resulted from Flood tectonism, especially in shallow seas (Snelling 2009).
Sea Level Rise and Death of Terrestrial Vertebrates
The flood continued forty days on the earth. The waters increased and bore up the ark, and it rose high above the earth. The waters prevailed and increased greatly on the earth, and the ark floated on the face of the waters. And the waters prevailed so mightily on the earth that all the high mountains under the whole heaven were covered. The waters prevailed above the mountains, covering them fifteen cubits deep. (Genesis 7:17–20 ESV)
They were eating and drinking and marrying and being given in marriage, until the day when Noah entered the ark, and the flood came and destroyed them all. (Luke 17:27 ESV)
Sea level rise has been associated with volcanism and tectonism (Schopf 1980; Tucker 1992). Supercontinent rifting is inferred to have created a large number of epicontinental seas (shallow seas lying upon continental areas) at low latitudes, enabling deep phosphorus-rich ocean waters to be moved into shallow shelfal environments (Donnelly et al. 1990).
Drowning of cratons has been inferred from uppermost Proterozoic and Cambrian strata. Peritidal carbonate platforms were drowned, to be followed in places by phosphorites and black shales (Brasier and Lindsay 2001). Evidence of sea level rise includes an upward fining sequence observed in Cambrian strata which has been interpreted as a deepening succession in locations such as the USA, Greenland, United Kingdom, Russia, Australia, Bolivia, and Ghana (Morton 1984). These occurrences include an upward fining sequence found exposed in the Grand Canyon’s Cambrian strata (Austin 1994). “Global or worldwide marine transgression” is the descriptor in the secular literature (Brasier 1982; Cook and Shergold 1984; Matthews and Cowie 1979). This is another way of saying global flooding! Sea level rose during the Flood as substantial volumes of water from the fountains were added to the earth’s surface and as the new warmer basaltic oceanic crust rose isostatically (Austin et al. 1994). We believe that the drowning of cratons correlates with the time the Ark rose and floated on the waters.
Seismic stratigraphic studies indicate that Ordovician strata had the highest sea level of the Paleozoic (Haq and Schutter 2008). We consider this to signify a peaking of the Flood waters. Consistent with this is the decrease of 87Sr/86Sr ratio following the peak in Cambrian strata (see fig. 2) which has been explained by a drop in the rate of erosion, decrease in silicate weathering rate, and/or the influence of rift-related hydrothermal activity (Nicholas 1996). After the earth, with its mountains, was totally covered by water, rain would no longer impact the land and so there would not be the same degree of continental erosion, as described in the previous section “Continental erosion and mass flows.”
Blotting Out
And all flesh died that moved on the earth, birds, livestock, beasts, all swarming creatures that swarm on the earth, and all mankind. Everything on the dry land in whose nostrils was the breath of life died. He blotted out every living thing that was on the face of the ground, man and animals and creeping things and birds of the heavens. They were blotted out from the earth. Only Noah was left, and those who were with him in the ark. (Genesis 7:21–23 ESV)
The key Hebrew word “machah” (מחה) has a general meaning of destroy, as well as more specific meanings such as blot out or wipe away. Other meanings of “machah” include erase as from a book, removing the memory of a people (such as the Amalekites), blotting out sins, and to wipe as one wipes a dish (Fouts and Wise 1998). Further aspects of the meaning for the word “machah” and which are relevant to the effects of the Flood fountains include:
- full of marrow (Strong 2007)—this is quite relevant as vertebrate bones contain marrow.
- abrasion—to stroke or rub; by implication to erase; also to smooth (Strong 2007).
- washing—erasing writing from a scroll was normally effected by washing (Van Gemeren 1997).
Land vertebrates, including man, have nostrils which admit and expel air for respiration in conjunction with the mouth. The blotting out process meant the death of all land vertebrates in the Flood (Genesis 7:23). We believe that these vertebrates had three different fates—dissolution, decomposition, and burial.
Many terrestrial vertebrate bones (as well as invertebrate shells) would have dissolved, particularly in near surface acidic waters, in the vicinity of the Flood’s volcanic fountains. This fate is the particular focus of this paper. The vertebrates close to the Flood fountains were not annihilated in the sense of going to nothing. Instead some evidence remained (Fouts and Wise 1998). When the pH of sediment solutions drop below 7, bone mineral will rapidly dissolve (Berna, Matthews, and Weiner 2004). Thus acidic volcanic waters would dissolve bones. In the vicinity of the active fountains of the great deep, the blotting out process is inferred to include dissolution of vertebrate skeletal phosphate. Widespread development of nutrient-enriched waters during the Precambrian-Cambrian transition has been indicated (Brasier and Lindsay 2001). δ13C is a measure of the ratio of the two stable isotopes of carbon—13C and 12C.3 Organic matter preferentially takes up the lighter isotope 12C. δ13C varies as a function of biological productivity and organic carbon burial. The Precambrian-Cambrian transition throughout much of Asia is marked by a sharp change in the carbon-isotope ratio δ13C suggesting that “As major phosphogenic events straddle this turning point in δ13C, huge fluxes in nutrient supply may have occurred . . .” (Brasier 1990a, 1990b; Cook 1992). The death and dissolution of terrestrial vertebrates in the Flood waters would have contributed greatly to this nutrient supply!
Another fate of land vertebrates would have been decomposition of corpses in surface waters. Mass mortality, and a sudden decrease in biological productivity, prior to the “Cambrian explosion” (the lowermost appearance of shelly fossils in the stratigraphic record) has been inferred from a negative δ13C geochemical anomaly in carbonate-containing black shale which is found around the globe at the Precambrian-Cambrian transition. It has been claimed that this corresponds to the widespread development of an oxygen-deficient shallow marine environment (Kimura and Watanabe 2001)—a “Strangelove ocean” (Hsu et al. 1985). We suggest that this relates to the consumption of oxygen in shallow waters due to the presence of bloated, decomposing corpses of drowned animals. This would have occurred in areas further away from the effects of the acidic Flood fountains since putrefying bacteria are sensitive to acid, and acidic waters delay the onset of putrefaction (Weigelt 2009). Hydrogen forms during vertebrate decomposition and captures oxygen from the surroundings (Weigelt 2009), creating anoxic conditions.
Away from the effects of the acidic fountains, many vertebrates would have suffered a third fate—rapidly buried by sediment and subsequently fossilized. Terrestrial vertebrate fossils are found in Upper Paleozoic and overlying strata (Tree of Life Web Project 2008).
Phosphorites Precipitate
The chemical conditions under which bone can recrystallize in place as phosphate are restricted and lie in a narrow alkaline pH range (Berna, Matthews, and Weiner 2004). At greater distances from the fountains, including shallow shelfal areas, ocean environments would likely have been more alkaline. This would have enabled precipitation of dissolved bones4 to contribute to amorphous phosphorite deposits around the Precambrian-Cambrian transition. Every continent except Antarctica is known to have Precambrian-Cambrian transition phosphorites (Cook 1992). Deposits known to have billions of tons of phosphorite are found in northwest Queensland, Vietnam, China, Mongolia, Russia, and Kazakhstan (Cook and Shergold 1984; Notholt, Sheldon, and Davidson 1989). In addition, significant deposits are found in Africa and South America (Cook 1992) (see fig. 3).
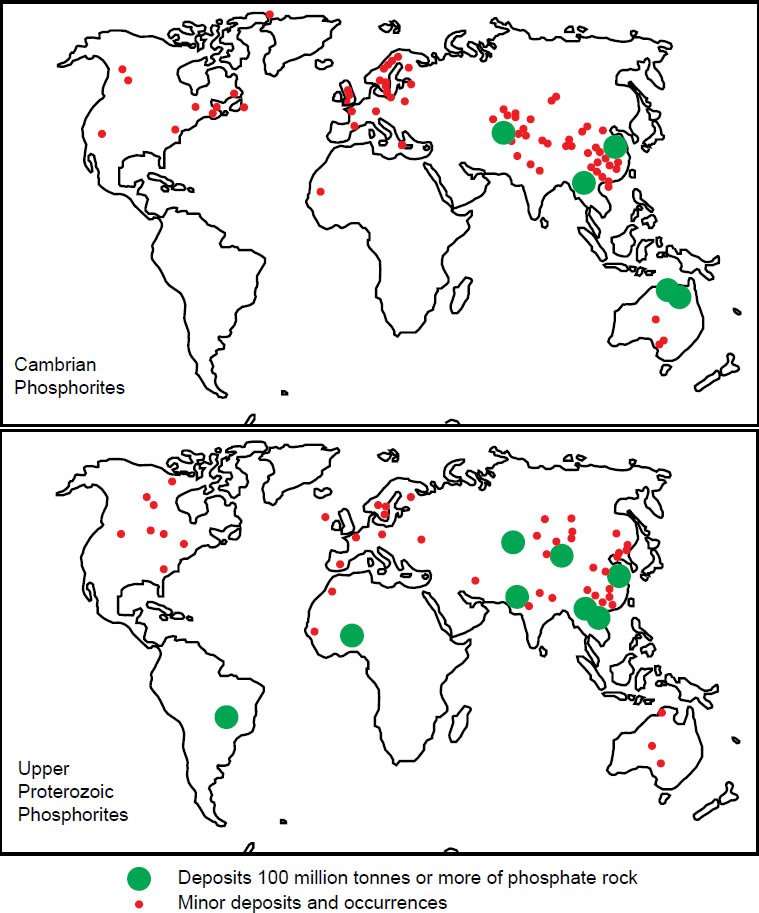
Fig. 3. Worldwide distribution of Precambrian-Cambrian transition phosphorite deposits (after Cook 1992). Cook’s lower map was labelled Precambrian phosphorites but these phosphorites are more specifically Upper Proterozoic phosphorites.
The phosphorite is associated with a worldwide marine transgression, and catastrophic ocean water mixing (deep anoxic and shallow oxic oceanic waters) as inferred from sulphur isotopes (Cook 1992; Cook and Shergold 1984). Upwelling of hydrogen sulphide-rich deep ocean water to become surface waters at the Precambrian-Cambrian transition is indicated by the basal Cambrian black shale molybdenum isotope signal (Wille et al. 2008). The Flood fountains of the great deep bursting forth would have caused catastrophic mixing of deep and shallow waters.
We infer that during the Flood, dissolved phosphate from vertebrate bones was moved by currents away from the fountains to shelfal areas. Shoreward transport and entrapment of phosphate grains in shallow nearshore zones may have helped form high-grade phosphate deposits (Cook and Shergold 1984). Bacteria may have had a role in the precipitation of phosphorite deposits under anoxic conditions in numerous locations around the world. Petrographic evidence has confirmed the presence of bacterial structures, including microbial mats (Nathan et al. 1993). Phosphate abundance in sediments may correlate with the abundance and activity of large sulphur bacteria (Schulz and Schulz 2005).
Paleomagnetism indicates mainly equatorial latitudes for Upper Proterozoic phosphorites (Cook and McElhinny 1979) as well as for Upper Proterozoic mixtites (Arnaud, Halverson, and Shields-Zhou 2011).
In numerous locations around the world, there is a general upward stratigraphic trend of Upper Proterozoic mixtites followed by phosphorites. This succession is mappable in many locations (Cook 1992; Ross 2013). Walther’s Law of the Correlation of Facies states that the vertical succession of facies reflects lateral changes in environment (Middleton 1973). Conversely, it states that when a depositional environment “migrates” laterally, sediments of one depositional environment come to lie on top of another. A classic example of this law is the vertical stratigraphic succession that typifies marine transgressions (Stanley 1999). Thus Upper Proterozoic mixtites may have formed in deeper water than the phosphorites as the sea transgressed landwards during the Noahic Flood.
Case Study—Northwest Queensland, Australia
Northwest Queensland is very significant in a global context for its economic importance as a world-class mineral province. The following provides a biblical framework for the chemical processes inferred in the formation of the region’s giant metallic ore deposits together with the adjacent huge phosphorite deposits.
The principal structural divisions in northwest Queensland are the Proterozoic Mount Isa Orogen and the mainly Paleozoic Georgina Basin (Geological Survey of Queensland 2012). Fig. 4 is a map which shows numerous metallic ore deposits of the Mount Isa Orogen together with the many sedimentary phosphate deposits of the Georgina Basin on the margin of the Mount Isa Orogen (Geological Survey of Queensland 2012). We consider that these metallic ore deposits and phosphate deposits formed in association with activity by the fountains of the great deep. We infer that the fountains of the great deep occurred at sites of breakup of the Rodinia supercontinent. This would include in the region of eastern Australia, with its Mount Isa Orogen.
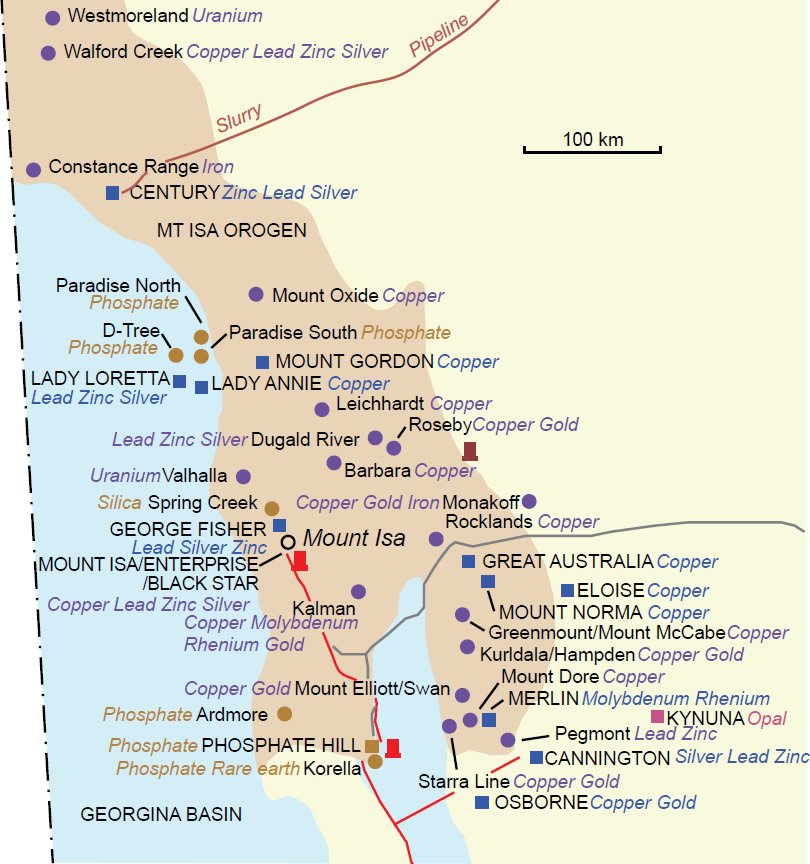
Fig. 4. Map of northwest Queensland showing metallic ore deposits of the Mount Isa Orogen and phosphorite deposits of the adjacent Georgina Basin (after Geological Survey of Queensland 2012).
Mount Isa Orogen
Mount Isa Orogen rocks outcrop over an area of some 50,000 km2 (19,305 mi2), roughly centered on the town of Mount Isa. This orogen consists of mainly marine sediments, felsic and mafic volcanics, granites and mafic intrusives (Blake 1987; Palfreyman 1984).
The Mount Isa Orogen is a world-class base metals province and contains giant sediment-hosted orebodies. These orebodies include Mount Isa (copper, lead, zinc, and silver), Cannington, Hilton, Century, George Fisher North, and Lady Loretta (lead, zinc, and silver), and Ernest Henry (copper and gold) (Lyons et al. 2006; Palfreyman 1984). Sediment-hosted silver-lead-zinc orebodies generally comprise accumulations of sulphide and sulphate minerals interbedded with anoxic and sulphidic marine sediments (Jell 2013).
These major stratiform zinc-lead-silver deposits exhibit many similar geological features. Host rocks are organic-rich black siltstone/shales (interpreted as formerly seafloor mud) that formed under anoxic conditions. The deposits are located adjacent to several major regional faults (Large et al. 2005). These faults likely acted as conduits for transport of hot metalliferous sedimentary brines from deep in the basin system. Laterally extensive strata-bound iron-manganese carbonate halos indicate significant volumes of hydrothermal fluids have interacted with seafloor and sub-seafloor sediments. Numerical modelling demonstrates that the deposits probably formed by free convection of marine waters and basinal brines during basin rifting events (Large et al. 2005).
Sedimentary exhalative deposits hosted in northern Australia’s Proterozoic rocks formed from relatively oxidized fluids that encountered reduced conditions at the site of mineralization (Lyons et al. 2006). In addition, seawater is alkaline enough to induce instability in metal complexes (Tarling 1981).
We infer that the relatively oxidized hot acidic oreforming fluids were flowing in association with active Noahic Flood fountains of the great deep. Interaction with anoxic, cool, reduced deep seafloor sediments, and more alkaline seawater made it possible for base metal sulfides to be deposited on the seafloor. Rapid ore formation is inferred from lead isotopes and from the rapid temperature drop as plumes carrying metals entered the sea (Snelling 1984). However, in near surface acidic waters close to the fountains, vertebrate bones would have dissolved as described previously in the “Blotted out” section of this paper.
Georgina Basin
The Georgina Basin is a large sedimentary basin straddling the Queensland/Northern Territory border. The basin’s area is approximately 330,000 km2 (127,413 mi2) of which about 144,000 km2 (55,598 mi2) is in Queensland. The Georgina Basin contains sediments that range from Upper Proterozoic to Lower Paleozoic and are in excess of 4500 m (14,763 ft) in thickness. Basin fill consists mainly of Cambrian and Ordovician marine sedimentary rocks— essentially carbonate rocks with minor sandstone and siltstone (Jell 2013). Anomalous geochemical signals, including trace metal enrichment, have been recorded in Cambrian rocks of the Georgina Basin (Donnelly, Shergold, and Southgate 1988). This is consistent with the idea that at a distance from the fountains there was still some slight evidence of the fountains activity.
Northwest Queensland’s known phosphate rock resources of 3 billion tons lie in large phosphorites hosted by Cambrian rocks in the Georgina Basin (Jell 2013). Mixtites such as the Little Burke “Tillite” and the Mount Birnie Beds are found stratigraphically below the phosphorites in the basin (Jell 2013; Palfreyman 1984).
Extensive phosphorite deposits are mined at Phosphate Hill. This deposit is located 140 km (87 mi) south-southeast of Mount Isa. It is a world-class rock phosphate resource that is close to the surface and easy to access and mine. This deposit is one of a generally north-south, 6 km (3.7 mi) long string of similar deposits distributed over an area of some 180 km2 (69 mi2). The phosphorite is hosted by the Cambrian Beetle Creek Formation (so named due to the presence of abundant trilobites) sequence. This sequence is in a 30 km (18 mi) wide by 100 km (62 mi) long, north-south elongated graben within the southern Mount Isa Orogen. Most of the phosphorite occurs in particulate form as pellets and minor replacement of fossil fragments, commonly as inclusions in the pellets (Porter 2011).
We infer that vertebrate bones were dissolved in acidic waters associated with Flood fountains in the region of the Mount Isa Orogen and that dissolved bones helped form the phosphorite deposits of the Georgina Basin.
Conclusion
In localities around the globe, great volumes of phosphorite in massive beds are found stratigraphically around the Precambrian–Cambrian transition, and generally above Upper Proterozoic mixtites.
The breaking up of the fountains of the great deep that triggered the Flood was accompanied by enormous rainfall. This caused immense runoff from the pre-Flood land surfaces, resulting in mass flows down slopes from land into the seas to form Upper Proterozoic mixtites.
Terrestrial vertebrate bones (and invertebrate shells) were dissolved under acidic conditions in the vicinity of the Flood fountains. However, at greater distances from the fountains the ocean chemistry was more normal, that is, slightly alkaline. This resulted in the dissolved bones being precipitated on adjacent shelfal areas contributing to amorphous phosphorite deposits around the Precambrian- Cambrian transition, and stratigraphically above Upper Proterozoic mixtites.
Chemical processes would have affected the pre-Flood world in the vicinity of the Flood fountains. These processes would have left their signature in the form of the distribution of minerals seen today. It is hoped that this paper may encourage further work to improve and refine our understanding of chemical processes in space and time in areas where the Flood’s fountains may have been active.
References
Allen, P. A., and P. F. Hoffman. 2005. “Extreme Winds and Waves in the Aftermath of a Neoproterozoic Glaciation.” Nature 433 (7022): 123–27.
Arnaud, E., G. P. Halverson, and G. Shields-Zhou. eds. 2011. The Geological Record of Neoproterozoic Glaciations. Geological Society Memoir No. 36. London, United Kingdom: Geological Society of London.
Austin, S. A. 1994. “A Creationist View of Grand Canyon Strata.” In Grand Canyon: Monument to Catastrophe. Edited by S. A. Austin, 57–82. Santee, California: Institute for Creation Research.
Austin, S. A., J. R. Baumgardner, D. R. Humphreys, A. A. Snelling, L. Vardiman, and K. P. Wise. 1994. “Catastrophic Plate Tectonics: A Global Flood Model of Earth History.” In Proceedings of the Third International Conference on Creationism. Edited by R. E. Walsh, 609–21. Pittsburgh, Pennsylvania: Creation Science Fellowship.
Austin, S. A., and K. P. Wise. 1994. “The Pre-Flood/Flood Boundary: As Defined in Grand Canyon, Arizona and Eastern Mojave Desert, California.” In Proceedings of the Third International Conference on Creationism. Edited by R. E. Walsh, 37–47. Pittsburgh, Pennsylvania: Creation Science Fellowship.
Bergeron, L. 1997. “Deep Waters.” New Scientist 155 (2097): 22–26.
Berna, F., A. Matthews, and S. Weiner. 2004. “Solubilities of Bone Mineral from Archaeological Sites: The Recrystallization Window.” Journal of Archaeological Science 31 (7): 867–82.
Berner, R. A. 1990. “Atmospheric Carbon Dioxide Levels Over Phanerozoic time.” Science 249 (4975): 1382–86.
Berner, R. A. 2006. “GEOCARBSULF: A Combined Model for Phanerozoic Atmospheric O2 and CO2.” Geochimica et Cosmochemica Acta 70 (23): 5653–64.
Berner, R. A., A. C. Lasaga, and R. M. Garrels. 1983. “The Carbonate-Silicate Geochemical Cycle and Its Effect on Atmospheric Carbon-Dioxide Over the Past 100 Million Years.” American Journal of Science 283 (7): 641–83.
Blake, D. H. 1987. Geology of the Mount Isa Inlier and Environs, Queensland and Northern Territory. Bulletin 225. Canberra, Australia: Bureau of Mineral Resources, Geology and Geophysics.
Brasier, M. D. 1982. “Sea Level Changes, Facies Changes and the Late Precambrian–Early Cambrian Evolutionary Explosion.” Precambrian Research 17 (2): 105–23.
Brasier, M. D. 1990a. “Nutrients in the Early Cambrian.” Nature 347 (6293): 521–22.
Brasier, M. D. 1990b. “Phosphogenic Events and Skeletal Preservation Across the Precambrian-Cambrian Boundary Interval.” In Phosphorite Research and Development. Edited by A. J. Notholt and I. Jarvis. Geological Society of London, Special Publication 52: 289–303.
Brasier, M. D. 1992. “Global Ocean-Atmosphere Change Across the Precambrian-Cambrian Transition.” Geological Magazine 129 (2): 161–68.
Brasier, M. D., and J. F. Lindsay. 2001. “Did Supercontinent Amalgamation Trigger the ‘Cambrian Explosion’?” In Ecology of the Cambrian Radiation. Edited by R. Riding and A. Zhuravlev, 69–89. New York, New York: Columbia University Press.
Cook, P. J. 1992. “Phosphogenesis Around the Proterozoic- Phanerozoic Transition.” Journal of the Geological Society of London 149 (4): 615–20.
Cook, P. J., and M. W. McElhinny. 1979. “A Re-Evaluation of the Spatial and Temporal Distribution of Sedimentary Phosphate Deposits in the Light of Plate Tectonics.” Economic Geology 74 (2): 315–30.
Cook, P. J., and J. H. Shergold. 1984. “Phosphorus, Phosphorites and Skeletal Evolution at the Precambrian- Cambrian Boundary.” Nature 308 (5956): 231–36.
Cook, P. J., and J. H. Shergold, 2005. Phosphate Deposits of the World. Vol. 1. Proterozoic and Cambrian Phosphorites. London, United Kingdom: Cambridge University Press.
Derry, L. A., M. D. Brasier, R. M. Corfield, A. Y. Rozanov, and A. Y. Zhuraylev. 1994. “Sr and C Isotopes in Lower Cambrian Carbonates from the Siberian craton: A Paleoenvironmental Record During the ‘Cambrian Explosion’.” Earth and Planetary Science Letters, 128 (3–4): 671–81.
Dickens, H., and A. A. Snelling. 2008. “Precambrian Geology and the Bible: A Harmony.” Journal of Creation 22 (1): 65–72.
Donnelly, T. H., J. H. Shergold, and P. N. Southgate. 1988. “Anomalous Geochemical Signals from Phosphatic Middle Cambrian Rocks in the Southern Georgina Basin, Australia.” Sedimentology 35 (4): 549–70.
Donnelly, T. H., J. H. Shergold, P. N. Southgate, and C. J. Barnes. 1990. “Events Leading to Global Phosphogenesis Around the Proterozoic/Cambrian Boundary.” Geological Society of London, Special Publications 52 (1): 273–87.
Evans, D. A. D. 2013. “Reconstructing Pre-Pangean Supercontinents.” Geological Society of America Bulletin 125 (11–12): 1735–51.
Faure, G., and J. L. Powell. 1972. Strontium Isotope Geology. Berlin, Germany: Springer-Verlag.
Fouts, D. M., and K. P. Wise. 1998. “Blotting Out and Breaking Up: Miscellaneous Hebrew Studies in Geocatastrophism.” In Proceedings of the Fourth International Conference on Creationism. Edited by R. E. Walsh, 217–28. Pittsburgh, Pennsylvania: Creation Science Fellowship. Geological Survey of Queensland. 2012. Queensland’s Mineral, Petroleum and Energy Operations and Resources Map. June 2012.
Hallam, A. 1981. Facies Interpretation and the Stratigraphic Record. Oxford, England: W. H. Freeman and Co.
Halverson, G. P., M. T. Hurtgen, S. M. Porter, and A. S. Collins. 2009. “Neoproterozoic-Cambrian Biogeochemical Evolution.” In Neoproterozoic-Cambrian Tectonics, Global Change and Evolution: A Focus on Southwestern Gondwana. Edited by C. Gaucher, A. N. Sial, G. P. Halverson, and H. E. Frimmel. Developments in Precambrian Geology 16: 351–65.
Haq, B. U., and S. R. Schutter. 2008. “A Chronology of Paleozoic Sea-Level Changes.” Science 322 (5898): 64–68. Hoffman, P. F., A. J. Kaufman, G. P. Halverson, and D. P. Schrag. 1998. “A Neoproterozoic Snowball Earth.” Science 281 (5381): 1342–46.
Hsu, K. J., H. Oberhänsli, J. Y. Goa, S. Shu, C. Haihong, and U. Krähenbühl. 1985. “‘Strangelove Ocean’ Before the Cambrian Explosion.” Nature 316 (6031): 809–11.
Ilyin, A. V. 1990. “Proterozoic Supercontinent, Its Latest Precambrian Rifting, Breakup, Dispersal Into Smaller Continents, and Subsidence of Their Margins: Evidence from Asia.” Geology 18 (12): 1231–34.
Jell, P. A. ed. 2013. Geology of Queensland. Brisbane, Australia: Geological Survey of Queensland.
Kimura, H. and Y. Watanabe. 2001. “Oceanic Anoxia at the Precambrian-Cambrian Boundary.” Geology 29 (11): 995–98.
Knoll, A. H., and M. R. Walter. 1992. “Latest Proterozoic Stratigraphy and Earth History.” Nature 356 (6371): 673–78.
Kump, L. R., S. L. Brantley, and M. A. Arthur. 2000. “Chemical Weathering, Atmospheric CO2, and Climate.” Annual Review of Earth and Planetary Sciences 28 (1): 611–67.
Large, R. R., S. W. Bull, P. J. McGoldrick, S. Walters, G. M. Derrick, and G. R. Carr. 2005. “Stratiform and Strata- Bound Zn-Pb-Ag Deposits in Proterozoic Sedimentary Basins, Northern Australia.” Economic Geology 100th Anniversary Volume: 931–64.
Lyons, T. W., A. M. Gellatly, P. J. McGoldrick, and I. C. Kah. 2006. “Proterozoic Sedimentary Exhalative (SEDEX) Deposits and Links to Evolving Global Ocean Chemistry.” In Evolution of Early Earth’s Atmosphere, Hydrosphere, and Biosphere—Constraints from Ore Deposits. Edited by S. F. Kesler and H. Ohmoto. Geological Society of America Memoir 198: 169–84.
Macdonald, G. A. 1972. Volcanoes. Englewood Cliffs, New Jersey: Prentice-Hall.
Martin, J. W. 2007. Concise Encyclopedia of the Structure of Materials. Oxford, United Kingdom: Elsevier. Matthews, S. C., and J. W. Cowie. 1979. “Early Cambrian Transgression.” Journal of Geological Society 136 (2): 133–35.
McGee, K. A., M. P. Doukas, R. Kessler, and T. M. Gerlach. 1997. Impacts of Volcanic Gases on Climate, the Environment, and People. U.S. Geological Survey Open-File Report, 97- 262. Reston, Virginia: U.S. Geological Survey.
McKenzie, N. R., N. C. Hughes, B. C. Gill, and P. M. Myrow. 2014. “Plate Tectonic Influences on Neoproterozoic-Early Paleozoic Climate and Animal Evolution.” Geology 42 (2): 127–30.
Middleton, G. V. 1973. “Johannes Walter’s Law of the Correlation of Facies.” Geological Society of America Bulletin 84 (3): 979–88.
Morton, G. R. 1984. “Global, Continental and Regional Sedimentation Systems and Their Implications.” Creation Research Society Quarterly 21 (1): 23–33.
Nathan, Y., J. M. Bremner, R. E. Loewenthal, and P. Monteiro. 1993. “Possible Active Role of Bacteria in Phosphorite Genesis.” Israel Geological Society Annual Meeting 1993, 93.
Nicholas, C. J. 1996. “The Sr Isotope Evolution of the Oceans During the ‘Cambrian Explosion’.” Journal of the Geological Society 153 (2): 243–54.
Notholt, A. J. G., R. P. Sheldon, and D. F. Davidson. eds. 1989. Phosphate Deposits of the World. Vol. 2. Phosphate Rock Resources. Cambridge, United Kingdom: Cambridge University Press.
Oard, M. J. 1997. “A Classic Tillite Reclassified as a Submarine Debris Flow.” Creation Ex Nihilo Technical Journal 11 (1): 7.
Palfreyman, W. D. 1984. Guide to the Geology of Australia. BMR Bulletin 181. Canberra, Australia: Australian Government Publishing Service.
Pearson, D. G., F. E. Brenker, F. Nestola, J. McNeill, L. Nasdala, M. T. Hutchison, S. Matveev, et al. 2014. “Hydrous Mantle Transition Zone Indicated by Ringwoodite Included within Diamond.” Nature 507 (7491): 221–24.
Peters, S. E., and R. R. Gaines. 2012. “Formation of the ‘Great Unconformity’ as a Trigger for the Cambrian Explosion.” Nature 484 (7394): 363–66.
Pisarevsky, S. A., J. B. Murphy, P. A. Cawood., and A. S. Collins. 2008. “Late Neoproterozoic and Early Cambrian Palaeogeography: Models and Problems.” In West Gondwana: Pre-Cenozoic Correlations Across the South Atlantic Region, Geological Society of London, Special Publication 294. Edited by R. J. Pankhurst, R. A. J. Trouw, B. B. de Brito Neves, and M. J. de Wit, 9–31. Bath, United Kingdom: The Geological Society Publishing House.
Porter Geoconsultancy Database. 2011. Georgina Basin— Phosphate Hill, Duchess—Queensland, Australia.
Pough, F. H., J. B. Heiser, and W. N. McFarland. 1989. Vertebrate Life. New York, New York: McMillan Publishing Company.
Roehl, P. O., and P. W. Choquette. eds. 1985. Carbonate Petroleum Reservoirs. Berlin, Germany: Springer-Verlag. Ross, M. R. 2013. “A Developing Schism in Flood Geology.” Journal of Creation 27 (3): 46–47.
Sawkins, F. J. 1983. “Tectonic Controls of the Time-Space Distribution of Some Proterozoic Metal Deposits.” Geological Society of America Memoirs 161: 179–90.
Schermerhorn, L. J. G. 1974. “Late Precambrian Mixtites: Glacial and/or NonGlacial?” American Journal of Science 274 (7): 673–824.
Schmandt, B., S. D. Jacobsen, T. W. Becker, Z. Liu, and K. G. Dueker. 2014. “Dehydration Melting at the Top of the Lower Mantle.” Science 344 (6189): 1265–68.
Schopf, T. J. M. 1980. Paleoceanography. Cambridge, Massachusetts: Harvard University Press.
Schulz, H. N., and H. D. Schulz. 2005. “Large Sulfur Bacteria and the Formation of Phosphorite.” Science 307 (5708): 416–18.
Shields, G. A. 2005. “Neoproterozoic Cap Carbonates: A Critical Appraisal of Existing Models and the Plumeworld Hypothesis.” Terra Nova 17 (4): 299–310.
Sigler, R., and V. Wingerden. 1998. “Submarine Flow and Slide Deposits in the Kingston Peak Formation, Kingston Range, Mojave Desert, California: Evidence for Catastrophic Initiation of Noah’s Flood.” In Proceedings of the Fourth International Conference on Creationism. Edited by R. E. Walsh, 487–501. Pittsburgh, Pennsylvania: Creation Science Fellowship.
Sigurdsson, H., B. Houghton, S. R. McNutt, H. Rymer, and J. Stix. 2015. The Encyclopedia of Volcanoes. Philadelphia, Pennsylvania: Elsevier.
Snelling, A. A. 1984. “The Recent, Rapid Formation of the Mount Isa Orebodies During Noah’s Flood.” Ex Nihilo 6 (3): 40–46.
Snelling, A. A. 2009. Earth’s Catastrophic Past: Geology, Creation and the Flood. Dallas, Texas: Institute for Creation Research.
Stanley, S. M. 1999. Earth System History, 134. New York: W. H. Freeman and Company.
Strong, J. 2007. Strong’s Exhaustive Concordance of the Bible. Peabody, Massachusetts: Hendrickson Publishers, Inc. Tarling, D. H. ed. 1981. Economic Geology and Geotectonics. Oxford, United Kingdom: Blackwell Scientific Publications.
Tree of Life Web Project. 2008. “Terrestrial Vertebrates.” www.tolweb.org/Terrestrial Vertebrates/14952.pdf.
Tucker, M. E. 1992. “The Precambrian–Cambrian Boundary: Seawater Chemistry, Ocean Circulation and Nutrient Supply in Metazoan Evolution, Extinction and Biomineralization.” Journal of the Geological Society 149 (4): 655–68.
Van Gemeren, W. A. ed. 1997. New International Dictionary of Old Testament Theology and Exegesis. Grand Rapids, Michigan: Zondervan.
Veizer, J., and W. Compston. 1974. “87Sr/86Sr Composition of Seawater During the Phanerozoic.” Geochimica et Cosmochimica Acta 38 (9): 1461–84.
Veizer, J., D. Ala, K. Azmy, P. Bruckkshen, D. Buhl, F. Bruhn, G. A. F. Carden et al. 1999. “87Sr/86Sr, δ13C and δ18O Evolution of Phanerozoic Seawater.” Chemical Geology 161 (1–3): 59–88.
Weigelt, J. 2009. Recent Vertebrate Carcasses and Their Paleobiological Implications. Chicago, Illinois: University of Chicago Press.
Wille, M., T. F. Nägler, B. Lehmann, S. Schröder, and J. D. Kramers. 2008. “Hydrogen Sulphide Release to Surface Waters at the Precambrian/Cambrian Boundary.” Nature 453 (7196): 767–69.
Wingerden, V. 2003. “Initial Flood Deposits of the Western Northern American Cordillera: California, Utah and Idaho.” In Proceedings of the Fifth International Conference of Creationism. Edited by R. L. Ivey Jr., 349–58. Pittsburgh, Pennsylvania: Creation Science Fellowship.
Xu, L., B. Lehmann, M. Jingwen, Q. Wenjun, and D. Andao. 2011. “Re-Os Age of Polymetallic Ni-Mo-PGE-Au Mineralization in Early Cambrian Black Shales of South China—A Reassessment.” Economic Geology 106 (3): 511–22.
Young, G. M. 1995. “Are Neoproterozoic Glacial Deposits Preserved on the Margins of Laurentia Related to Fragmentation of Two Supercontinents?” Geology 23 (2): 153–56.