The views expressed in this paper are those of the writer(s) and are not necessarily those of the ARJ Editor or Answers in Genesis.
Abstract
North America’s Proterozoic geology provides evidence for enormous erosion and the formation of extensive water flow systems, even spanning the continent. It is proposed that these processes may be correlated with the early Creation Week and early Flood.
The emergence of land on Day Three implies the formation of a topographic gradient from land to the sea, and associated water movement, on land and under sea, with immense runoff from the newly emergent land. This article infers that what is now southeastern North America became a region of pre-Flood mountains.
Immediate pre-Flood topography was drastically changed by the erosional effect of the Flood’s prolonged and globally extensive rain. Even high mountains were not spared. This article infers that today’s Grenville, Mazatzal, and Yavapai provinces in southeastern North America represent the roots of mountains eroded in the Flood.
Keywords: Proterozoic, North America, tectonism, erosion, water flows, sedimentation, Creation Week, Flood, Grenville, zircon
Introduction
The case has been put that a number of North American Proterozoic sedimentary sequences formed when enormous water flow systems ensued as major tectonic events took place in areas to the east of the sedimentary basins (Rainbird, Cawood, and Gehrels 2012). The Grenvillian and Pan-African tectonic Events have been claimed to be the largest such events in the earth’s geological history, based on detrital zircon age data derived from river sands (Rino et al. 2008). These continent-scale events may be correlated with the Bible.
In order to make continent-scale sedimentary models of early Creation Week and early Flood more tangible, there is considered to be a need for young-earth creationist (YEC) geoscience research to better identify provinces that were eroded even on a continent-scale, and to infer first order current directions resulting from such enormous water flows.
The Grenvillian Event is associated in secular literature with Mesoproterozoic thickening of continental crust. In striking contrast to most of North America’s older Proterozoic provinces, the lithosphere of southeastern North America has an overall northeast-southwest regional trend. This includes the Late Paleoproterozoic Yavapai and Mazatzal provinces, as well as the Mesoproterozoic Grenville province. It is proposed that formation of these provinces, along with North America’s Grenvillian Event, be correlated with processes in the earlier part of Day Three.
The Grenvillian Event is inferred to correlate with the building of high mountains and emergence of land on Day Three of Creation Week (Snelling 2009). It is inferred that in addition to the formation of crystalline rocks on Day Three, some synorogenic Mesoproterozoic sediments also formed since the emergence of land on Day Three would have fostered water flow, erosion, and transport of detritus downslope towards the sea.
The Pan-African Event is associated in secular literature with Neoproterozoic rifting of the Rodinian supercontinent, including the Cordilleran and Appalachian margins of North America. In this article the Pan-African Event is inferred to have initiated with the breaking open of the fountains of the great deep. Catastrophic plate tectonics have been used for a global Noahic Flood model of earth history (Austin et al. 1994).
Stupendous rain of Noah’s Flood would have triggered immense erosion of all the pre-Flood land and consequent deposition of huge volumes of sediment. Grenvillian-age detrital zircons and early Neoproterozoic sandstone cross-bedding together provide evidence that mountain belts in the southeastern part of the North American continent contributed sedimentary detritus to enormous surface water flow systems that crossed to the west side of the North American continent, a distance of 3000 km (Rainbird, Cawood, and Gehrels 2012). Paleocurrents derived from cross-bedding in thick fluvial deposits in basins indicates regionally consistent west-northwesterly transport (Rainbird, Cawood, and Gehrels 2012). Along with tremendous erosion of subaerial continental landmasses, torrential rain would have caused huge submarine mass flows, especially on continental margins (Sigler and Wingerden 1998; Snelling 2009; Wingerden 2003) commonly interpreted as mid-Neoproterozoic (Cryogenian) “glacials”.
In this paper radiometric “ages” are used in a relative sense, and not absolute time. Ga refers to billion years ago radiometric “age”.
1. Geology Today
1.1 Precambrian crystalline basement provinces
Significant basement rock provinces and their approximate radiometric ages in North America include the southeastern Grenville (1.55–1.0 Ga), Yavapai (1.7–1.68 Ga) and Mazatzal (1.65–1.6 Ga) provinces, together with the adjacent more northern Trans-Hudson (1.9–1.8 Ga) and Superior (> 2.5 Ga) provinces (Fig. 1).
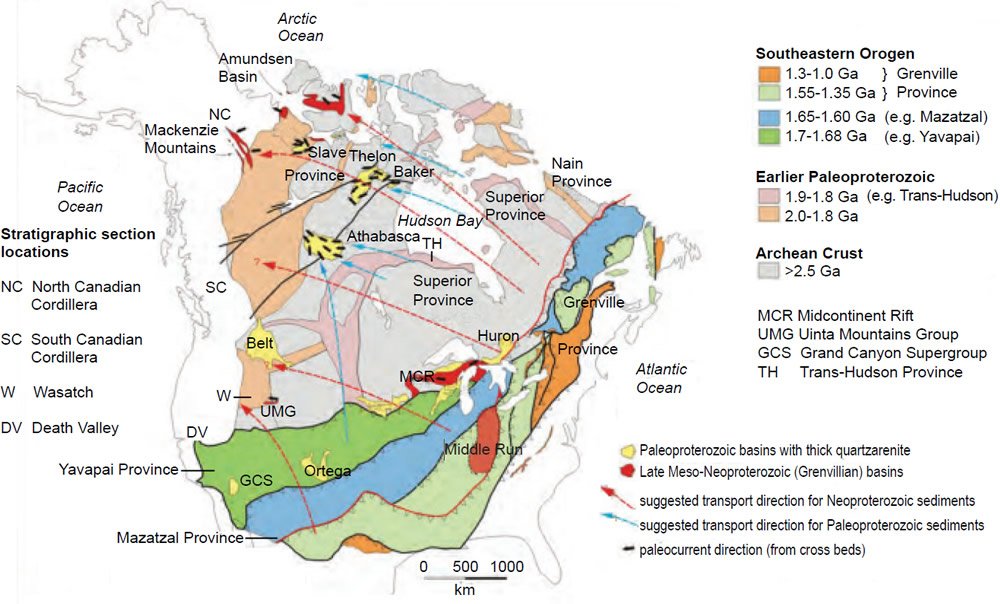
Fig. 1. Precambrian geological map of North America showing location of key Proterozoic basins and southeastern orogenic provinces. The Grenville Province has been interpreted as the roots of a deeply eroded mountain chain that formed from about 1.2 to 1.0 Ga during multi-stage continental growth. The proximal part of the Grenvillian depositional system includes deposits located in the Middle Run Basin and the Midcontinent Rift.
- Dashed red arrows illustrate proposed transport directions of huge water flow systems that crossed the continent, spreading northwesterly. Basement provinces in the southeastern part of the continent are inferred to have contributed sedimentary detritus to form the thick Neoproterozoic sandstone successions preserved in basins of western North America (for example, Amundsen Basin, Mackenzie Mountains, Uinta Mountains Group [UMG], and Grand Canyon Supergroup [GCS]). Detrital zircon grains that are commonly found there yield “ages” that are characteristic of the Grenville Province, several thousand kilometers away, on the other side of the continent.
- Dashed blue arrows show the suggested transport direction of Paleoproterozoic sediments, shed from the Yapavai and Trans-Hudson provinces (shown in green and pink, respectively, on the map) to thick sandstone successions of Paleoproterozoic basins such as the Athabasca and Thelon.
- Black arrows indicate paleocurrent directions interpreted from cross-bedding in thick fluvial deposits in Proterozoic basins in northern Canada. Regionally consistent west-northwesterly transport is indicated.
The location of stratigraphic sections on the western margin of North America (Fig. 3) are shown.
(Figure modified from Rainbird, Cawood, and Gehrels 2012)
In striking contrast to most of North America’s older Paleoproterozoic and Archean provinces, the lithosphere of southeastern North America has an overall northeast-southwest regional trend. This includes the Late Paleoproterozoic Yavapai and Mazatzal provinces, as well as the Mesoproterozoic Grenville Province. These northeast-trending provinces are characterized by voluminous granitoid plutonism (Mints 2015; Whitmeyer and Karlstrom 2007).
The Grenville Province is characterized by extremely high grades of metamorphism (amphibolite and granulite facies) in both basement terranes and supracrustal rocks of the Grenville Supergroup. Rock types are ensialic with quartzofeldspathic gneisses, migmatites and metasediments including marble (Davidson 1995; Jamieson et al. 2011). The Grenville Province commonly has moderate to high-grade metamorphic rocks outcropping at the surface today. In the type area for the Grenville Province (eastern Canada and the State of New York), most of its rocks are greatly deformed and consist of upper amphibolite to granulite facies metamorphic minerals (Gower, Kamo, and Krogh 2008).
There is a Grenvillian age peak in geochemical and isotopic signatures, identified in North America, Western Australia, and global data sets, spanning a quarter of the globe, or a distance of approximately 20,000 km long and as wide as 800 km, including a core zone several hundred kilometers wide (Van Kranendonk and Kirkland 2013).
The Trans-Hudson Province consists of low metamorphic grade sedimentary-volcanic complexes (Mints 2007). The Trans-Hudson Province is the largest and best exposed Paleoproterozoic belt in North America (Zhao et al. 2002). This belt has Archean provinces on both sides.
Archean provinces include the Superior Province, which is the world’s largest such province. These provinces essentially consist of high metamorphic grade granulite-gneiss terranes and low-grade granite-greenstone terranes (Mints 2007) (Fig. 1).
1.2 Earlier Proterozoic sedimentary sequences
Western North America
The Mesoproterozoic Unkar Group of the southwestern United States consists of limestone, shale, quartzite, sandstone, and lavas (Timmons et al. 2001). All units within the Unkar Group contain a complex distribution of Paleoproterozoic zircons typically between 1.8 and 1.6 Ga, with large age peaks at ca. 1.45 Ga, and young populations between 1.3 and 1.2 Ga (Mulder et al. 2017). Mudstone petrology studies and interbedded sandstone detrital zircon geochronology indicate that Unkar mudstones were derived largely from the southeastern orogenic region of North America (including the Grenville Province of southwest Texas and the adjacent Yavapai-Mazatzal terranes) (Bloch et al. 2006).
The Mesoproterozoic Belt-Purcell Basin contains an 18 to 20 km thick sedimentary succession dominated by fine-grained terrigenous facies, including marine turbidites with tholeiitic sills (Lydon 2007). The lower part of the succession contains abundant distinctive 1.6–1.5 Ga detrital zircon populations not characteristic of southeast North America. Upper Belt-Purcell strata contain ca. 1.73 Ga detrital zircon age populations that match the signature of metasedimentary rocks of the Yavapai Province to the south and southeast (Jones, Daniel, and Doe 2015).
Northern Canada
The widespread, relatively flat-lying upper parts of the Paleoproterozoic Thelon and Athabasca basins successions in northern Canada are dominated by hematite-stained quartz arenites (Palmer, Kyser, and Hiatt 2004). The middle and upper sequences of the Thelon Basin contain Archean age detrital zircons. A 3.4 Ga zircon population cannot be correlated to the immediately surrounding basement (Palmer, Kyser, and Hiatt 2004).
The Paleoproterozoic Burnside River Formation, of the Kilohigok Basin in Arctic Canada is dominated by sandstone and overlies Archean granite-greenstone basement rocks of the Slave Province (Ielpi et al. 2015).
1.3 Neoproterozoic sedimentary sequences
Neoproterozoic sedimentary cover is found in both the western (Cordilleran) and eastern (Appalachian) margins of North America, as well as cratonic sequences (Frazier and Schwimmer 1987). These Neoproterozoic deposits are mainly immature clastic sediments, with lesser volcanic rocks, and include mixtites which are commonly interpreted to be “glacial” (Hoffman 1989).
Western North America
Northwestern Canada’s Amundsen Basin and Mackenzie Mountain areas contain Grenvillian age zircons (Rainbird et al. 1997; Rainbird and Young 2009). Detrital zircon geochronology of the Amundsen Basin’s Shaler Supergroup enables potential correlation with strata from the Ogilvie and Mackenzie platforms (Rainbird, Jefferson, and Young 1996; Rainbird et al. 1997). The Nelson Head Formation within the Shaler Supergroup has cross-bedded sandstone (Fig. 2).
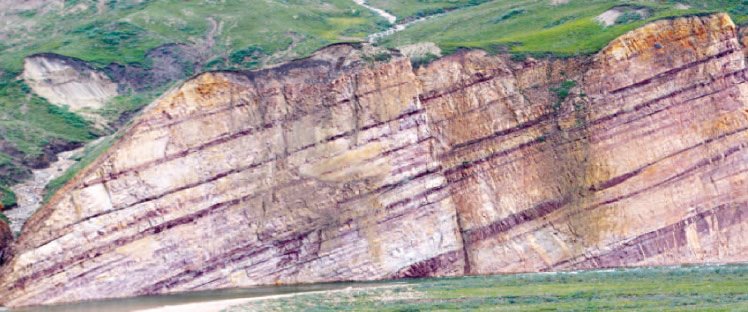
Fig. 2. Distant western end of the Grenvillian river system represented by braided-type deposits of the Shaler Supergroup, Amundsen Basin, Canada. The exposed section has a thickness of about 35 m (from Ielpi and Rainbird 2016a). The Shaler Supergoup can be correlated with other earlier Neoproterozoic sequences such as the Little Dahl/ Fifteenmile Group of the North Canadian Cordillera (Macdonald et al. 2010) (Fig. 3).
Regional lithostratigraphic correlation of early Neoproterozoic Sequence B and Fifteen Mile Group, (Fig. 3) on the northwest margin of North America includes a laterally continuous, 0.5–2 km thick, quartzarenite marker. The vast majority (85%) of the zircons in this sequence are of Mesoproterozoic age, with a high fraction clustering between 1.25–1 Ga, and this closely matches the age of synorogenic intrusions of the Grenville Province (Rainbird et al. 1997).
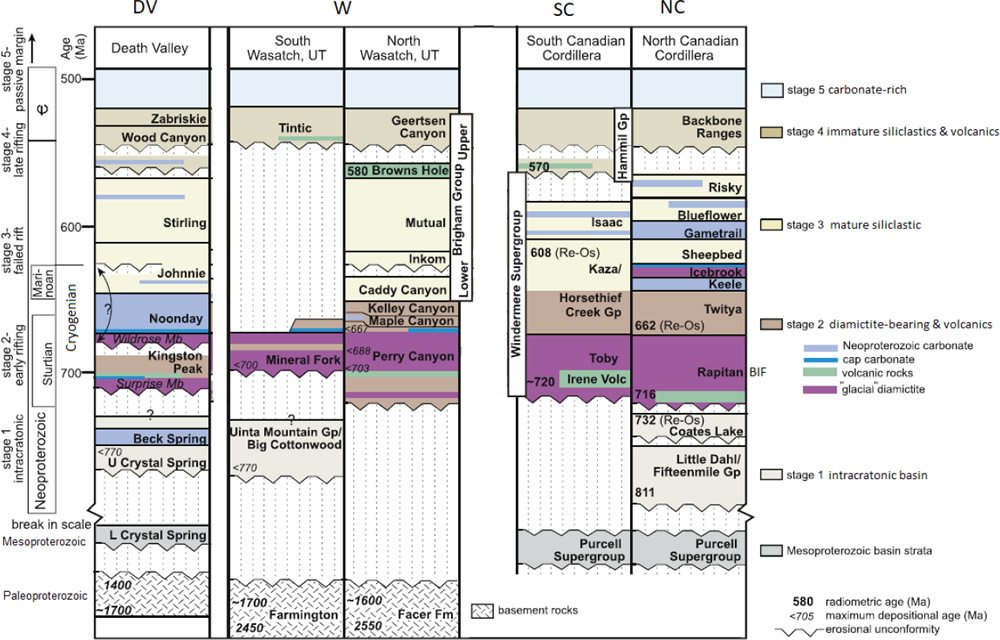
Fig. 3. Reference stratigraphic sections and published age constraints for Neoproterozoic to Cambrian strata along the Cordilleran margin (see Fig. 1 locations of sections). Stratigraphic sections range from Paleoproterozoic basement rocks upwards to Cambrian carbonate-rich strata. Data is from Death Valley, Wasatch, and the Canadian Cordillera. Interpreted rifting and “glacial” episodes are indicated (Yonkee et al. 2014).
Grenville-age detrital zircon comprises a significant proportion of most sedimentary successions in western North America, including Neoproterozoic successions of southwestern USA (Rainbird, Cawood, and Gehrels 2012). In the southwestern United States the Grand Canyon Supergroup in effect represents a “Time gap of ~1.2 Ga” in the ‘Great Unconformity’ between the Paleozoic (~0.5 Ga sandstone) and the Paleoproterozoic basement (including ~1.75 Ga gneiss) (Timmons, Karlstrom, and Dehler 1999). The Grand Canyon Supergroup essentially consists of the Neoproterozoic Chuar Group and the underlying Mesoproterozoic Unkar Group sedimentary sequences (Timmons et al. 2001).
Detrital zircon geochronology of Neoproterozoic and lowermost Cambrian rocks from sedimentary basins located along the western margin of North America, and now exposed mainly in the central Cordillera of California and Nevada have yielded detrital zircons with characteristic “Grenvillian” ages (Fedo, Sircombe, and Rainbird 2003).
The correlation of Uinta Mountain (Utah), Pahrump (Death Valley) and Chuar (Grand Canyon) strata (Fig. 1) of southwestern USA is supported by similar patterns in detrital zircon ages, provenance, biostratigraphy, and, to some extent, C-isotope stratigraphy. (Dehler et al. 2017). The Kingston Peak Formation within the Pahrump Group contains mixtites which are commonly said to be “glacial” (Fig. 3).
Southeastern North America
There are a number of early Neoproterozoic deposits proximal to the Grenville Province, including the Middle Run Formation (Fig. 1), which contains Grenvillian detrital zircons (Krabbendam et al. 2017). The Midcontinent Rift (Fig. 1) has a very thick succession of basaltic lavas (15–20 km) and overlying early Neoproterozoic sedimentary rocks (Allen et al. 2015; Hoffman 1989; Krabbendam et al. 2017).
2. Inferred Geological History
2.1 Zircon geochronology
Zircons contain high concentrations of important trace elements, including the U-Pb radiogenic isotope system which is highly suited to high-precision U-Pb geochronology. Dates from individual zircon grains typically reflect the magmatic crystallization age (and also metamorphism) of a crystalline basement rock (Hawkesworth et al. 2010).
Because zircon is highly refractory and resilient at earth’s surface, it occurs in virtually all sedimentary deposits and so provides a critical link in understanding the provenance of sediments (Fedo, Sircombe, and Rainbird 2003). Sedimentary rocks contain eroded and transported detritus derived from precursor igneous, metamorphic, and sedimentary material. The age composition of detrital zircons within such sedimentary rocks provides an indication of crystalline source rock regions and so input to sedimentary basins (Spencer and Kirkland 2016). Since zircons are by nature resistant they may undergo multiple recycling events in sedimentary deposits (Rainbird, Cawood, and Gehrels 2012).
2.2 Early Creation Week versus early Flood
The first three days of Creation Week may be seen as a fashioning of an initial ex nihilo-created earth (Dickens and Snelling 2008).
Strontium isotope trends of the Neoproterozoic indicate enormous continental erosion (Peters and Gaines 2012) (Fig. 4) and I infer that this can be correlated with the erosion of land associated with the Flood’s rain (Dickens 2016). The corollary of this is that underlying Archean to Mesoproterozoic crystalline basement and sedimentary rocks can be correlated with early Creation Week. The pre-Flood earth surface was destroyed (Genesis 6:13) in the sense of being totally wiped or eroded away (Matthew 24:39). It is considered that immense erosion of the land and related abrasion was not favorable for preservation of fossils. It was only once the sea transgressed the land (“Cambrian transgression” [Matthews and Cowie 1979]) that macrofossils had a chance to be formed and preserved.
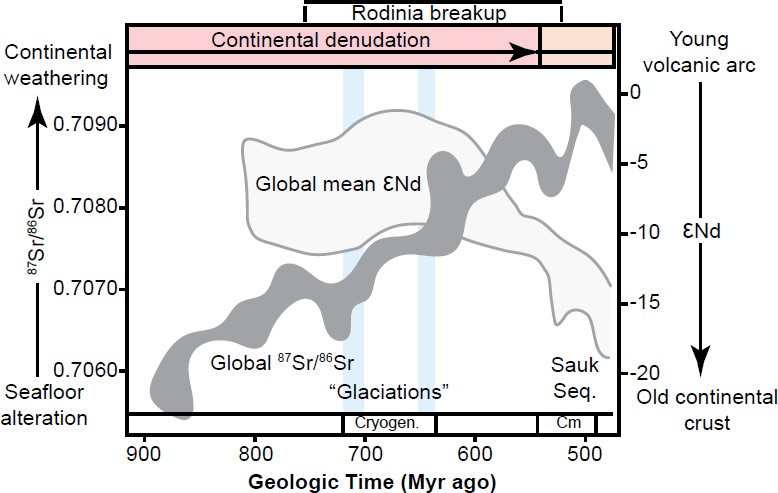
Fig. 4. Summary of major geochemical and sedimentary patterns derived from Neoproterozoic to Cambrian strata (modified from Peters and Gaines 2012):
- 87Sr is a radiogenic daughter isotope of 87Rb and is found in silicate rocks such as granite. The abundance of radiogenic 87Sr relative to “common” 86Sr in a sample of sediment is related to the amount of sediment that originated from erosion of continental crust as opposed to that originating from the ocean. The observed increase in Neoproterozoic strontium isotope ratios 87Sr/86Sr has been explained by accelerated rates of erosion.
- The subsequent decline in 87Sr/86Sr ratio in post Cambrian strata indicates greater oceanic influence and a time of accumulation of sediments on the continents as more of the Sauk Sequence began to be deposited, reducing the direct erosive impact of rain on landmasses.
- High rates of erosion of basin hinterlands can also explain the very negative neodymium isotope ratio εNd(t) in the Cambrian. A more negative εNd(t) is related to fractionation of neodymium isotopes observed in crustal rocks compared to a standard.
I infer that:
- the erosion of land associated with the Flood’s rain can be correlated with Neoproterozoic sequences (including mixtites interpreted as mass flows rather than as “glacials” and that Sr isotope trends indicate continental erosion). The radiometric “timespan” for the Neoproterozoic to Cambrian increase in 87Sr/86Sr ratio is approximately 0.4 Ga, but in the biblical framework the actual time elapsed would have been of the order of weeks to months.
- a corollary is that Archean to Mesoproterozoic rocks can be correlated with early Creation Week.
- the pre-Flood land surface was destroyed (Genesis 6:13) in the sense of being totally wiped away (eroded) (Matthew 24:39).
- the subsequent decline in 87Sr/86Sr ratio in post Cambrian strata is due to the presence of a globe-covering ocean so that the rain no longer directly impacted the land.
Braided-type deposits are found in the earlier Neoproterozoic sequences whereas “glacial” deposits are found in the mid-Neoproterozoic (Cryogenian) sequences.
2.3 Water flow episodes
Braided rather than meandering river systems have been described as characteristic of the Precambrian (Eriksson et al. 2013). The lack of channelized, meandering (low energy) river systems and the presence of mature quartz-arenite sandstone bodies (Rainbird, Cawood, and Gehrels 2012) is consistent with extremely high energy and relatively short duration (days) for global early Flood and early Creation Week geological processes. These are subaerial sheet-braided deposits and not submarine landslide (mass flow) deposits. Sheet-braided deposits mainly consist of sandstone whereas mass flow deposits consist of poorly sorted sediments. The high energy and immense erosion of these episodes did not require deep time. The Flood, likely removed evidence of any pre-Flood meandering river channels.
A very different hydrologic regime compared to today’s rivers is indicated for these sheet-braided deposits since they have a relatively high percentage of coarse sediments and there is little evidence for the presence of deep channels (Rainbird, Cawood, and Gehrels 2012). The sources of detritus for some modern large river systems, such as the Amazon and Mississippi, is mainly local (Iizuka et al. 2005; Mapes et al. 2004). However, the Grenvillian belt-sourced detritus may have been deposited up to thousands of kilometers away (Rainbird, Cawood, and Gehrels 2012). Although the Amazon has a high water flow, it has meanders indicating relatively low energy flow.
By measuring the inclination direction of crossbeds formed by migration of underwater dunes, the way ancient water flowed can be inferred (Rainbird and Young 2009). It has been inferred that “braided river system of pan-continental proportions that may have emanated from sources along the entire 5000+ km length of the Grenville orogenic front” (Rainbird et al. 1997). The water flow may have been a laterally extensive sheet flow regime, rather than just braided river systems.
The hypotheses of “big-river” systems and the presence of a craton scale fluvial blanket in Neoproterozoic and Early Cambrian times is supported by the successful integration of sedimentological information, such as paleocurrent analysis, with U-Pb geochronology (Fedo, Sircombe, and Rainbird 2003). The configuration of sandstones in Neoproterozoic basins of northwestern Canada provides evidence for laterally extensive sheet-braided flow, indicative of high energy. Amalgamated, fluvial sheet sandstones in the stratigraphic record are often interpreted as the deposits of braided rivers (McLaurin and Steel 2007).
Not just the Flood, but the Creation Week would also have had great movements of water! There was a global ocean on Days One and Two, and land only emerged on Day Three. Separation of land from the sea may be consistent with sufficient continental crust growth that land emerged. This would have resulted in downslope water flow draining newly emergent land and downslope water currents in the sea.
It has been proposed that several large intracontinental sedimentary basins of late Paleoproterozoic to Mesoproterozoic age are remnants of a regional sand blanket deposited unconformably on crystalline rocks of the Canadian Shield by a series of large river systems (Rainbird, Cawood, and Gehrels 2012). The sedimentology, sequence stratigraphy and basins’ fill depositional age are similar and can be correlated between basins that are thousands of kilometers apart. Similar westerly paleocurrent directions have been interpreted for these depocenters. These observations and those from Neoproterozoic sequences led to the suggestion that the preserved sedimentary basins were the remnants of enormous river systems that emerged when several major regional tectonic Events (such as the Hudsonian and Grenvillian) were taking place in areas to the east of the sedimentary basins (Rainbird, Cawood, and Gehrels 2012; Young 1978). Such conditions and relationships existed several times during the development of the ancient North American craton (Rainbird, Cawood, and Gehrels 2012).
2.4 Precambrian basement provinces and early Creation Week
And God said, “Let the waters under the heavens be gathered together into one place, and let the dry land appear.” And it was so. God called the dry land earth, and the waters that were gathered together he called Seas. And God saw that it was good. (Genesis 1:9–10 ESV)
the one who by his strength established the mountains, being girded with might. (Psalm 65:6 ESV)
Southeastern crystalline basement provinces
Massive river systems have been interpreted to have formed when major mountain building (the Grenvillian thermal-tectonic Event) occurred on the eastern side of the North American continent. (Rainbird, Cawood, and Gehrels 2012). In addition, major rift and aulocogen-related basins were formed in the Mesoproterozoic such as the Midcontinent Rift Basin and the Belt-Purcell Basin (Pirajno 1992).
It has been claimed that as Proterozoic provinces developed, they underwent deformation and thickening but without the major fragmentation, widespread dispersion and collision of continents typical of the post-Permian (Hamilton 2011). This is consistent with the idea of the progressive formation of only one supercontinent by Day Three, which later broke up in Noah’s Flood. I propose that by Day Three the supercontinent had thickened sufficiently for dry land to emerge and that formation of late Paleoproterozoic and Mesoproterozoic provinces of the southeast of the North American craton, along with North America’s Grenvillian Event, be correlated with processes in the earlier part of Day Three.
The rocks of the Grenville Province in the southeast of the North American craton have been interpreted to represent the roots of a deeply eroded mountain chain (Rainbird, Cawood, and Gehrels 2012). I propose that the rocks of today’s Grenville Province represent the eroded roots of pre-Flood high mountains (Genesis 7:19) which had formed on Day Three. Many Bible translations refer to high mountains in Genesis 7:19 (for example, ESV, NIV, NASB, TLV, YLT98). Strong’s concordance has mountain or range of hills. Within the Grenville Province the common occurrence of high-grade (amphibolite to granulite facies) metamorphic rocks at the earth’s surface today indicates uplift and erosion of tens of kilometers of crust since the bulk of mountain building was complete (Rainbird, Cawood, and Gehrels 2012).
Thermodynamic constraints on pressure-temperature stability of metamorphic mineral assemblages of gneisses and migmatites have been used to infer tens of kilometers depth of formation (Jamieson et al. 2011; Rivers 2015). Using Grenvillian examples, metamorphic petrologist Wynn-Edwards maintained that the occurrence on all scales of folds with similar style (that is, thick hinges and thin limbs), together with coaxial refolding, were signs of extreme ductility during deformation. (Rivers 2015).
The Grenvillian tectonic Event has been described as “Perhaps the greatest orogenic (mountain-building) event in earth’s history . . .” (Rainbird, Cawood, and Gehrels 2012, 584). Tectonic analysis and paleogeographic reconstructions indicate that the Grenvillian orogen was possibly the longest and widest in earth history, extending for a quarter of the globe, or a distance of approximately 20,000 km long and as wide as 800 km, including a core zone several hundred kilometers wide (Van Kranendonk and Kirkland 2013).
The 1.2–1.1 Ga radiometric “age” peak in oxygen isotope and incompatible trace element signatures, identified in North America, Western Australia, and globally, indicate that the Grenvillian orogeny may represent a unique episode in earth history (Van Kranendonk and Kirkland 2013). Oxygen isotopes (δ18O) in zircon grains and incompatible trace element (ITE) (Zr, Th) concentrations in magmatic rocks are used as proxies to test for variations in the degree of crustal recycling through geological time (Van Kranendonk and Kirkland 2013). ITEs are preferentially excluded from common rock-forming minerals during fractional crystallization due to their unsuitable size and/or charge to fit within the cation sites of crystals, and are partitioned into silicate liquids during melting. Elevated concentrations of Zr and Th above crustal values in magmas reflect enhanced crustal recycling and the importance of preexisting continental crust in the orogenic cycle. Oxygen isotopes are also elevated toward heavy values with crustal recycling. The significant peak in both ITEs and oxygen isotopes at 1.2–1.1 Ga (during the Grenvillian Event) is consistent with a time of episodic crustal growth.
The mountains melt like wax before the Lord, before the Lord of all the earth. (Psalm 97:5 ESV)
The Grenville Province has been described as a large hot orogen (Rivers 2015). Geothermometry (based on metamorphic mineral assemblages) of upper amphibolite- and granulite-facies gneisses in the Grenville hinterland revealed temperatures in the range 700 ± 50°C or higher, although subsequently shown to commonly underestimate peak temperature due to post-peak resetting. Enhanced radioactive decay has been considered a possible factor in such heating (Clark et al. 2011).
The Grenville Province is known for its complex ductile gneissic structure, high grade of metamorphism, and polyphase reworking, features indicative of residence in the deep crust (Rivers 2015). Field relations indicate that crust underwent melt-enhanced ductile flow laterally for tens of kilometers (Jamieson et al. 2011) and thus erosion of tens of kilometers of crust. The record of this erosion was expected to be preserved in the vestiges of a pan-Rodinian drainage system that would have delivered huge volumes of detritus to adjacent basins, located across the interior of North America (Fedo, Sircombe, and Rainbird 2003).
The northeast-trending Yavapai, Matzatzal, and Grenville provinces are characterized by voluminous granitoid plutonism (Mints 2015; Whitmeyer and Karlstrom 2007) and considerable thickening of continental crust (Van Kranendonk and Kirkland 2013). It is proposed that the growth and huge crustal thickening of late Paleoproterozoic and Mesoproterozoic provinces of the southeast of the North American craton (Fig. 1), along with the Grenvillian orogeny, were processes from Day Three when land emerged for the first time. Only by very late Neoproterozoic or early Paleozoic time do significant indicators of subduction (and thus “true” plate tectonics) appear, including complete ophiolites and high-pressure, low-temperature metamorphism (Stern 2005). Thus plate tectonics may not have existed in the Archean to Mesoproterozoic. This is consistent with the idea of the growth of only one supercontinent by Day Three.
Northern Paleoproterozoic and Archean crystalline basement provinces
Based on the paleomagnetic record, an interpretation has been made that the Precambrian continental crust was essentially intact from the Archean until late Neoproterozoic rifting (Piper 2015). This interpretation may be consistent with the idea of the progressive growth of only one supercontinent from Day One to the earlier part of Day Three.
Provinces such as the Superior Province may have together formed part of one late Archean supercraton (Condie et al. 2017). The large late Archean zircon U-Pb age peak is thought to represent coincidental cooling within individual provinces (Percival 2004) and the beginning of stable cratons (Frazier and Schwimmer 1987). The Paleoproterozoic Trans-Hudson Province is believed to have involved internal rifting and reworking of Archean crust, rather than collision between two Archean continental blocks (Mints 2015).
2.5 Earlier Proterozoic sedimentation and early Creation Week
The sea is his, for he made it, and his hands formed the dry land. (Psalm 95:5 ESV)
Western Northern America
The combination of mudstone petrology and interbedded sandstone detrital zircon geochronology affords insights into the provenance, hydrodynamic effects, weathering regime, and diagenesis of the Mesoproterozoic Unkar Group. It has been concluded that Unkar mudstones were derived largely from the Grenville Province of southwest Texas and the adjacent Yavapai-Mazatzal provinces (Bloch et al. 2006). All units within the Unkar Group contain a complex distribution of Paleoproterozoic zircons. (Mulder et al. 2017) This may be a result of water flowing from mountains formed on Day Three in North America’s southeastern region. Unkar Group and other Late Mesoproterozoic strata in southwestern North America have been inferred to indicate north-flowing continental-scale rivers sourced from the developing Grenville Orogen.
The Belt-Purcell Basin (Fig. 1 Belt and Fig. 3) is considered to have been filled by marine and fluviatile sediments (Lydon 2007) and this may be consistent with formation in a pre-Flood sea. The ca. 1.47–1.45 Ga lower Belt-Purcell strata contains abundant distinctive non-Laurentian 1.61–1.50 Ga detrital zircon populations. Exotic cratonic sources such as Australia have been inferred! However, upper Belt-Purcell strata contain strongly unimodal ca. 1.73 Ga detrital zircon age populations that match the detrital zircon signature of Paleoproterozoic metasedimentary rocks of the Yavapai Province to the south and southeast (Jones, Daniel, and Doe 2015).
Northern Canada
Provenance analysis using zircon grains has been used to infer that big water flow systems transported Paleoproterozoic sediments, shed from the Yapavai and Trans-Hudson Provinces to Paleoproterozoic basins of northern Canada such as the Athabasca and Thelon (Rainbird, Cawood, and Gehrels 2012). Such late Paleoproterozoic sediments may have resulted from erosion as continental crust thickened and land emerged on Day Three. The Thelon and Athabasca basins have been interpreted to have formed in a dominantly fluvial environment and from zircon provenance studies to include far-travelled detritus (Palmer, Kyser, and Hiatt 2004; Rainbird and Young 2009). Economic deposits of unconformity-related uranium occur in association with Paleoproterozoic basins such as the Athabasca Basin (Hanly et al. 2006). Unconformity on Archean crystalline basement rocks is consistent with inferred erosional action of Day Two water movement on Archean crystalline rocks that may have formed on Day One.
Outcrop evidence from Paleoproterozoic sediments of the Kilohigok Basin in Arctic Canada (such as the occurrence of incised paleovalleys and sandsheets with high width to thickness ratios) indicates deep-channeled drainage as well as sheet-braided rivers (Ielpi and Rainbird 2016b). “Glacial” deposits (and sheet-braided rivers) may have resulted from the great movements of waters during Day Two (Dickens and Snelling 2008).
2.6 Neoproterozoic sedimentation and early Flood
by his knowledge the deeps broke open, and the clouds drop down the dew. (Proverbs 3:20 ESV)
In the six hundredth year of Noah’s life, in the second month, on the seventeenth day of the month, on that day all the fountains of the great deep burst forth, and the windows of the heavens were opened. (Genesis 7:11 ESV)
Tectonism
The phrases, “deeps broke open” and “fountains of the great deep burst forth,” imply fragmentation of the earth’s crust. This is inferred to correlate with Neoproterozoic breaking up of the Rodinian supercontinent (Dickens and Snelling 2008). Worldwide simultaneous (commencing on the same day) erupting of springs or fountains (including eruption of volcanic material) was followed by global rainfall (Dickens and Snelling 2008). Rifting, volcanic and depositional stages in the Neoproterozoic have been inferred (Yonkee et al. 2014) (Fig. 3).
Assuming a configuration of Rodinia supercontinent like that in Fig. 5, it may be inferred that these high mountains were situated close to a site of Pan-African breakup east of the Grenville Province and thus Flood fountains which poured out rain. There are other configurations of Rodinia than that shown in Fig. 5, but one thing in common is that the eastern margin of North America is a site of breakup of Rodinia. This is considered to be correlatable with when the fountains of the great deep burst forth. Intense erosion of these high mountains may have been accentuated due to their proximity to such fountains and the ensuing rain. Reconstruction of the Rodinia supercontinent was based on recognition of correlatable Neoproterozoic rifted passive margins (Evans 2013). Catastrophic plate tectonics have been used for a global Noahic Flood model of earth history (Austin et al. 1994).
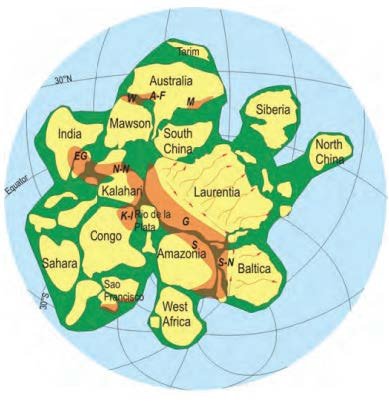
Fig. 5. One possible configuration of the supercontinent Rodinia. The Grenville Mountain Belt is shown in orange. Sediments have been interpreted to have been transported by an enormous system of braided rivers over a huge area of the supercontinent, as suggested by the arrows on Laurentia (the North American craton). G is the Southeastern Orogen shown in Fig. 1. (Rainbird, Cawood, and Gehrels 2012). There may be other interpreted configurations of Rodinia, but the Grenville Mountain Belt remains immediately adjacent to a region where Rodinia is inferred to have fragmented.
Worldwide continental rifting (and subsequent ocean formation beginning at 0.6 Ga) has been inferred (Hoffman 1989) and this has been described as the dismemberment of the Rodinia supercontinent “by the most important single continental break-up event in geological history shortly before the dawn of the Cambrian” (Bond et al. 1984; Piper 2009). The Pan-African thermal-tectonic Event is associated with this massive global rifting (Hoffman 1989). It has been postulated that episodic rifting events at the margins of North America between 0.8 and about 0.6 Ga record the fragmentation of a Neoproterozoic supercontinent (Bond, Christie-Blick, and Kominz 1984; Hoffman 1989). This is consistent with the initial breaking open of the crust with the bursting forth of the fountains of the great deep on a specific day, followed by rifting, further extension and ocean formation.
The final basin-forming episode of Proterozoic rocks involved a series of rift basins that once more preserve evidence of multiple “glaciations” and contain banded iron formations (Yeo 1981). The Rapitan-type iron formation of northwest Canada (NC of Figs. 1 and 4) is associated with Neoproterozoic mixtites, debris flow deposits, turbidites, and widespread flood basalts (Cox et al. 2013). Iron isotope values are consistent with oxidation of ferruginous waters during marine transgression and rift-related hydrothermal activity associated with the breakup of Rodinia is inferred (Cox et al. 2013). Remarkably similar Neoproterozoic “glacials” are found on the west and east margins of North America and this has been related to supercontinent fragmentation (Young 1995). Accommodation space for many “glacials” may have been provided by rifting. (Young 2013). A rift-related tectonostratigraphic model has been proposed for most of the Neoproterozoic diamictite-bearing successions (Eyles and Januszczak 2004; Schermerhorm 1974). Whatever the primary cause of the “glaciation” may have been, there is overwhelming evidence for a link with the Pan-African tectonic events (Deynoux et al. 2006).
Rain-triggered erosion
Behold, I will destroy them with the earth. (Genesis 6:13b ESV)
and rain fell upon the earth forty days and forty nights. (Genesis 7: 12 ESV)
and they were unaware until the flood came and swept them all away, so will be the coming of the Son of Man. (Matthew 24:39 ESV)
The mountains saw you and writhed; the raging waters swept on. (Habakkuk 3:10b ESV)
he who removes mountains, and they know it not, when he overturns them in his anger. (Job 9:5 ESV)
The Flood’s rain caused enormous erosion of the land, and at the same time the sea level rose, until eventually all the earth’s land was covered (Dickens 2016). It is important that the enormous erosion of land aspect of the Flood be recognized and not just the marine transgression aspect of the Flood. The pre-Flood surface topography was thoroughly destroyed (Genesis 6:13) by the Flood’s subaerial erosion and ensuing deposition. However, Creation Week crystalline basement would have remained though eroded. The destruction of the earth mentioned in Genesis 6:13 is not annihilation (going to nothing) but instead it is the total transformation of the surface of the earth, such that the pre-Flood world was no longer recognizable.
The initial erosional phase of Noah’s Flood correlates with the timing of the initial Pan-African continental breakup (~0.8 Ga [Hoffman 1989]) and with development of Neoproterozoic geology (Dickens and Snelling 2015). It is inferred that immense continental erosion (Peters and Gaines 2012) and enormous water flow systems occurred early in the Flood due to the rain impacting subaerial land (Dickens and Snelling 2015; Dickens 2016).
In addition to massive erosion of continental landmasses, enormous torrential rain would have eventually caused huge mass flows sweeping down from higher areas into the seas, depositing immense volumes of sediments. A modern analogy is Hawaii where there are giant undersea landslides and large debris fields on the seafloor (Ten Bruggencate 2001). In today’s world, heavy rainfall and earthquakes set off landslides in rugged areas (Bai et al. 2013). How much more so would earthquakes have occurred with rifting episodes during the Flood Event, thus setting off gigantic submarine landslides, now recognized as Neoproterozoic mixtites on the western and eastern margins of North America. Mid-Neoproterozoic (Cryogenian) mixtites, interpreted by secular scientists as occurring during “glaciations” are more likely submarine mass flow deposits (Schermerhorn 1974) formed in the rain stage of Noah’s Flood due to enormous water flow on the continents (Dickens 2016).
In the modern world, local downpours of rain persisting over a limited period can cause great devastation of property and habitats, loss of life, landslides, mud flows, erosion, and swollen rivers. A recent study has concluded that the magnitude of flooding in the USA is highest in regions which experience the most extraordinary precipitation, and that precipitation is the primary driver of floods. In addition, maximum peak discharges tend to occur in basins in close vicinity to the ocean that also have mountainous terrain (Saharia et al. 2017). The Bible describes the greatest rain event ever recorded—40 days and nights of rain falling on the whole earth (Genesis 7:12) and so the effects were vastly greater than for today’s floods which are restricted to local basins.
The Neoproterozoic Sr ratio indicator trend is consistent with immense erosion of the continents (Peters and Gaines 2012) (Fig. 4). The major 87Sr/86Sr isotope ratio increase between 0.9 Ga and 0.5 Ga is consistent with erosion of highly radiogenic continental crust (Dickens 2016; Dickens and Snelling 2015; Meert and Powell 2001; Peters and Gaines 2012) during the Neoproterozoic (Fig. 4).This evidence is consistent with what would be expected from the effects of enormous rainfall on the North American continent during the early Noahic Flood. Rubidium (Rb) is common in the crust’s silicate minerals. 87Sr is a radiogenic daughter isotope of 87Rb. Therefore the abundance of radiogenic 87Sr relative to “common” 86Sr in a sample of sediment is related to the amount of sediment that originated from continental crust as opposed to that originating from the ocean. Similarly, a negative εNd(t) is related to fractionation of neodymium isotopes observed in crustal rocks compared to a standard.
Continental denudation, enhanced chemical weathering and changes in global ocean chemistry are indicated by numerous geochemical signatures associated with the Great Unconformity (Peters and Gaines 2012). The evidence is consistent with what would be expected from the effects of enormous rainfall and on the North American continent during the early Noahic Flood (Dickens 2016). The sharp stratigraphic contact typically separates Precambrian crystalline basement from the quartz- and feldspar-rich marine sands of the basal Sauk Sequence (Sloss 1964). Exposure and chemical weathering of silicate minerals derived from continental basement rocks during the formation of the Great Unconformity exerted an important control on seawater chemistry and global biogeochemical cycling in several ways, including the consumption of atmospheric CO2 and the release of a number of types of ions to the oceans (Peters and Gaines 2012).
In the Grand Canyon, the Great Unconformity is said to represent about 1.2 Ga of “missing” radiometric time since 1.75 Ga gneiss is overlain directly by 0.5 Ga sandstone. Such locations where Paleozoic sediments lie directly on basement are considered to be where there was erosion only and not deposition of detritus eroded off of the land. The Grand Canyon Supergroup is considered to represent the “missing time” (Timmons, Karlstrom, and Dehler 1999). The Chuar Group of the Grand Canyon Supergroup may represent detritus derived from continental erosion. Subsequently the Grand Canyon’s Paleozoic sediments were deposited as the sea level rose sufficiently to then completely cover the land of the whole earth.
Western North America
The style of sedimentation would have varied in time and location as the Flood Event progressed (Fig. 3). In early Neoproterozoic basins of northwestern Canada, paleocurrent directions (Figs. 1 and 2) derived from cross-bedding in thick subaerial fluvial deposits indicate regionally consistent west-northwesterly transport. Detrital zircon grains commonly occur on the western side of the continent which give ages that are characteristic of the Grenville Province, several thousand kilometers away (Rainbird, Cawood, and Gehrels 2012). Neoproterozoic sandstone cross-bedding and detrital zircons together provide evidence that mountain belts in the southeastern part of the North American continent contributed sedimentary detritus to enormous river systems that crossed the continent, spreading northwesterly and forming the thick sandstone successions preserved in Neoproterozoic sedimentary basins of northwestern Canada. Some zircons may have been transported more than 3000 km. The lateral extent of the inferred fluvial system was at least 1200 km, much broader than any fluvial system on today’s earth (Rainbird et al. 2017).
Zircon grains of Grenvillian age were recovered from early Neoproterozoic sedimentary basins in northwestern Canada (including the Amundsen Basin), more than 3000 km away from the nearest probable source in the Grenville Province on the other side of the continent. In addition, paleocurrents derived from cross-bedding in thick fluvial deposits in these basins indicates regionally consistent west-northwesterly transport (Rainbird, Cawood, and Gehrels 2012). Braided rivers indicate high energy unlike meandering rivers. This is all evidence for enormous water flow systems as part of a gigantic erosional episode. In the USA Cordillera, thousands of kilometers to the south of northwestern Canada, there are correlative strata that show similar detrital zircon patterns. Such strata includes the Uinta Mountains Group and the Grand Canyon Supergroup. This provides further evidence for large-scale river systems, transporting detritus that were laterally extensive, originating from multiple sources along the great Grenville Mountain Belt (Rainbird, Cawood, and Gehrels 2012).
The Uinta Mountain Group of west-central USA formed with deposition of fluvial to marine, feldspathic to quartzose sandstone, conglomerate, and mudstone. Detrital zircon patterns indicate a mix of local basement sources to the north and distal sources to the southeast of North America. Subsequently, in association with rifting and volcanism, the Perry Canyon formation was deposited and included mixtites claimed to be “glacials” (Yonkee et al. 2014) (Figs. 1 and 3).
In areas such as the Grand Canyon, the Great Unconformity is the contact between overlying Paleozoic layered sediments and underlying, either Paleoproterozoic crystalline basement or tilted Grand Canyon Supergroup’s Neoproterozoic Chuar Group, sediments. Locations where Paleozoic sediments lie directly on basement are considered to be where there was erosion only and not deposition of detritus eroded off the land. The Neoproterozoic Chuar Group has been associated with breakup of the Rodinia supercontinent, whereas the underlying Mesoproterozoic Unkar Group has been associated with the “assembly” of Rodinia (Timmons, Karlstrom, and Dehler 1999). I infer respectively, early Flood detritus from rain’s erosion of continent, and Day Three emergence of land with thickening of crust and synorogenic intracratonic basin sedimentation.
Mid-Neoproterozoic (Cryogenian) “glacials” may instead be mass flow deposits formed with enormous submarine landslides during tectonic activity as the Flood progressed. Neoproterozoic mixtites of the North American Cordillera have been interpreted as submarine mass flow deposits (Sigler and Wingerden 1998; Snelling 2009; Wingerden 2003). The Kingston Peak Formation (Figs. 1 and 4) in California provides evidence for debris flows and catastrophic coarse clastic deposits in Noah’s Flood rather than glaciation (Sigler and Wingerden 1998). Neoproterozoic mixtites have an intimate association with sedimentary rocks indicating warm climates (Young 2013).
In addition, the Rapitan-type banded iron formations associated with such “glacials” in the Neoproterozoic may have formed hydrothermally in deeper water and in association volcanic activity (Pirajno 1992). A catastrophic pouring out of volcanics and associated banded iron formations in the Neoproterozoic is inferred to have occurred early in Noah’s Flood (Dickens 2017).
Southeastern North America
Evidence for significant rifting on the eastern (Appalachian) margin in southeastern North America has been identified (Frazier and Schwimmer 1987) and this was more or less simultaneous with an event of worldwide continental rifting (and subsequent ocean formation beginning at 0.6 Ga) (Hoffman 1989).
The upper Keweenawan of the Midcontinent Rift (Fig. 1) has Neoproterozoic sediments proximal to, and believed to be sourced from, the Grenville Orogen. (Krabbendam et al. 2017). This thick detrital sediment overlies volcanic fill (Lucas and St-Onge 1998). Water flow may have diverted into the rift. Other proximal early Neoproterozoic sequences containing detritus derived from the Grenville Orogen include the Middle Run Formation (Fig. 1), the Hazel Formation and the Flinton Formation (Krabbendam et al. 2017).
Conclusion
In striking contrast to most of North America’s older Proterozoic provinces, the lithosphere of southeastern North America has an overall northeast-southwest regional trend. This includes the Late Paleoproterozoic Yavapai and Mazatzal provinces, as well as the Mesoproterozoic Grenville Province. It is proposed that formation of late Paleoproterozoic and Mesoproterozoic provinces of the southeast of the North American craton, along with North America’s Grenvillian Event, be correlated with processes in the earlier part of Day Three.
The Grenvillian thermal-tectonic Event, associated with huge Mesoproterozoic crustal thickening, is inferred to correlate with Day Three emergence of land and high mountain-building. In addition to the formation of crystalline rocks on Day Three, some synorogenic Mesoproterozoic sediments also formed since the emergence of land on Day Three would have fostered water flow, erosion, and transport of detritus downslope towards the sea.
The Pan-African thermal-tectonic Event is consistent with an enormous erosional and depositional regime expected in the early stages of the Flood of Noah. It has been postulated that episodic rifting events at the margins of North America between 0.8 Ga and about 0.6 Ga record the fragmentation of the Neoproterozoic Rodinia. This is consistent with the initial breaking open of the crust with the bursting forth of the fountains of the great deep on a specific day, followed by rifting, volcanism, further extension, and eventually ocean formation.
The Flood’s rain caused enormous erosion of the land, and at the same time the sea level rose, until eventually all the earth’s land was covered. It is important that the enormous erosion of land aspect of the Flood be recognized and not just the marine transgression aspect of the Flood.
North America’s Grenville Province is inferred to be the roots of a deeply eroded pre-Flood mountain chain, which was situated just to the west today of a zone where fountains of the great deep later erupted and rain fell at the onset of the Flood. Intense erosion of Grenvillian high mountains may have been accentuated due to their proximity to Flood fountains and the ensuing rain and tectonism.
References
Allen, P. A., P. G. Erikkson, F. F. Alkmim, P. G. Betts, O. Catuneanu, R. Mazumder, Q. Meng, and G. M. Young. 2015. “Classification of Basins, With Special Reference to Proterozoic Examples.” In Precambrian Basins of India: Stratigraphic and Tectonic Context. Edited by R. Mazumder and P. G. Eriksson, Memoir 43: 5–28. London, United Kingdom: Geological Society.
Austin, S. A., J. R. Baumgardner, D. R. Humphreys, A. A. Snelling, L. Vardiman, and K. P. Wise. 1994. “Catastrophic Plate Tectonics: A Global Flood Model of Earth History.” In Proceedings of the Third International Conference on Creationism. Edited by R. E. Walsh, 609–621. Pittsburgh, Pennsylvania: Creation Science Fellowship.
Bai, S-B, C. Cheng, J. Wang, B. Thiebes, and Z. Zhang. 2013. “Regional Scale Rainfall- and Earthquake-Triggered Landslide Susceptibility Assessment in Wudu County, China.” Journal of Mountain Science 10 (5): 743–753.
Bloch, J. D., J. M. Timmons, L. J. Crossey, G. E. Gehrels, and K. E. Karlstrom. 2006. “Mudstone Petrology of the Mesoproterozoic Unkar Group, Grand Canyon, U.S.A.: Provenance, Weathering, and Sediment Transport on Intracratonic Rodinia.” Journal of Sedimentary Research 76 (9–10): 1106–1119.
Bond, G. C., P. A. Nickeson, and M. A. Kominz. 1984. “Breakup of a Supercontinent Between 625 Ma and 555 Ma: New Evidence and Implications for Continental Histories.” Earth and Planetary Science Letters 70 (2): 325–345.
Clark, C., I. C. W. Fitzsimmons, D. Healy, and S. L. Harley. 2011. “How Does the Continental Crust Get Really Hot?” Elements 7 (4): 235–240.
Condie, K. C., N. Arndt, A. Davaille, and S. J. Puetz. 2017. “Zircon Age Peaks: Production or Preservation of Continental Crust?” Geosphere 13 (2): 227–234.
Cox, G. M., G. P. Halverson, W. G. Minarik, D. P. Le Heron, F. A. Macdonald, E. J. Bellefroid, and J. V. Strauss. 2013. “Neoproterozoic Iron Formation: An Evaluation of Its Temporal, Environmental and Tectonic Significance.” Chemical Geology 362: 232–249.
Davidson, A. 1995. “A Review of the Grenville Orogen in Its North American Type Area.” AGSO Journal of Australian Geology and Geophysics 16 (1/2): 3–24.
Dehler, C., G. Gehrels, S. Porter, M. Heizler, K. Karlstrom, G. Cox, L. Crossey, and M. Timmons. 2017. “Synthesis of the 780–740 Ma Chuar, Uinta Mountain, and Pahrump (ChUMP) Groups, Western USA: Implications for Laurentia-Wide Cratonic Marine Basins.” Geological Society of America Bulletin 129 (5–6): 607–624.
Deynoux, M., P. Affaton, R. Trompette, and M. Villeneuve. 2006. “Pan-African Tectonic Evolution and Glacial Events Registered in Neoproterozoic to Cambrian Cratonic and Foreland Basins of West Africa.” Journal of African Earth Sciences 46 (5): 397–426.
Dickens, H. 2016. “The ‘Great Unconformity’ and Associated Geochemical Evidence for Noahic Flood Erosion.” Journal of Creation 30 (1): 8–10.
Dickens, H. 2017. “Banded Iron Formations Formed Rapidly.” Journal of Creation 31 (2): 14–16.
Dickens, H., and A. A. Snelling. 2008. “Precambrian Geology and the Bible: A Harmony.” Journal of Creation 22 (1): 65–72.
Dickens, H., and A. A. Snelling. 2015. “Terrestrial Vertebrates Dissolved Near Flood Fountains.” Answers Research Journal 8: 437–447.
Eriksson, P. G., S. Banerjee, O. Catuneanu, P. L. Corcoran, K. A. Eriksson, E. E. Hiatt, M. Laflamme, et al. 2013. “Secular Changes in Sedimentation Systems and Sequence Stratigraphy.” Gondwana Research 24 (2): 468–489.
Evans, D. A. D. 2013. “Reconstructing Pre-Pangean Supercontinents.” Geological Society of America Bulletin 125 (11–12): 1735–1751.
Eyles, N., and N. Januszczak. 2004. “‘Zipper-Rift’: A Tectonic Model for Neoproterozoic Glaciations During the Breakup of Rodinia after 750 Ma.” Earth-Science Reviews 65 (1–2): 1–73.
Fedo, C. M., K. N. Sircombe, and R. H. Rainbird. 2003. “Detrital Zircon Analysis of the Sedimentary Record.” Reviews in Mineralogy and Geochemistry 53 (1): 277–303.
Frazier, W. J., and D. R. Schwimmer. 1987. Regional Stratigraphy of North America. New York, New York: Plenum Press.
Gower, C. F., S. Kamo, and T. E. Krogh. 2008. “Indentor Tectonism in the Eastern Grenville Province.” Precambrian Research 167 (1–2): 201–212.
Hamilton, W. B. 2011. “Plate Tectonics Began in Neoproterozoic Time, and Plumes from Deep Mantle Have Never Operated.” Lithos 123 (1–4): 1–20.
Hanly, A. J., T. K. Kyser E. E. Hiatt, J. Marlatt, and S. Foster. 2006. “The Uranium Mineralization Potential of the Paleoproterozoic Sioux Basin and Its Relationship to Other Basins in the Southern Lake Superior Region.” Precambrian Research 148 (1–2): 125–144.
Hawkesworth, C. J., B. Dhuime, A. B. Pietranik, P. A. Cawood, A. I. S. Kemp, and C. D. Storey. 2010. “The Generation and Evolution of the Continental Crust.” Journal of the Geological Society, London 167 (2): 229–248.
Hoffman, P. F. 1989. “Precambrian Geology and Tectonic History of North America.” In The Geology of North America: An Overview. Edited by A. W. Bally and A. R. Palmer, 447– 512. Boulder, Colorado: Geological Society of America.
Ielpi, A. and R.H. Rainbird. 2016a. “Highly Variable Precambrian Fluvial Style Recorded in the Nelson Head Formation of Brock Inlier (Northwest Territories, Canada).” Journal of Sedimentary Research 86 (3): 199–216.
Ielpi, A., and R. H. Rainbird. 2016b. “Reappraisal of Precambrian Sheet-Braided Rivers: Evidence for 1.9 Ga Deep-Channelled Drainage.” Sedimentology 63 (6): 1550–1581.
Ielpi, A., R. H. Rainbird, J. W. Greenman, and C. G. Creason. 2015. “The 1.9 Ga Kilohigok Paleosol and Burnside River Formation, Western Nunavut: Stratigraphy and Gamma-Ray Spectrometry.” Summary of Activities, Canada-Nunavut Geoscience Office 1–10.
Iizuka, T., T. Hirata, T. Komiya, S. Rino, I. Katayama, A. Motoki, and S. Maruyama. 2005. “U-Pb and Lu-Hf Isotope Systematics of Zircons From the Mississippi River Sand: Implications for Reworking and Growth of Continental Crust.” Geology 33 (6): 485–488.
Jamieson, R. A., M. J. Unsworth, N. B. W. Harris, C. L. Rosenberg, and K. Schulmann. 2011. “Crustal Melting and the Flow of Mountains.” Elements 7 (4): 253–260.
Jones, J. V., C. G. Daniel, and M. F. Doe. 2015. “Tectonic and Sedimentary Linkages Between the Belt-Purcell Basin and Southwestern Laurentia During the Mesoproterozoic ca. 1.60–1.40 Ga.” Lithosphere 7(4):465–472.
Krabbendam, M., H. Bonsor, M. S. A. Horstwood, and T. Rivers. 2017. “Tracking the Evolution of the Grenvillian Foreland Basin: Constraints from Sedimentology and Detrital Zircon and Rutile in the Sleat and Torridon Groups, Scotland.” Precambrian Research 295: 67–89.
Lucas, S. B., and M. R. St-Onge. 1998. “Geology of the Precambrian Superior and Grenville Provinces and Precambrian Fossils in North America.” Geology of Canada, no. 7. Geological Survey of Canada.
Lydon, J. W. 2007. “Geology and Metallogeny of the Belt-Purcell Basin.” In Mineral Deposits of Canada: A Synthesis of Major Deposit Types, District Metallogeny, the Evolution of Geological Provinces, and Exploration Methods. Edited by W. D. Goodfellow, 581–607. Geological Association of Canada, Mineral Deposits Division, Special Publication 5.
Macdonald, F. A., M. D. Schmitz, J. L. Crowley, C. F. Roots, D. S. Jones, A. C. Maloof, J. V. Strauss, et al. 2010. “Calibrating the Cryogenian.” Science 327 (5970): 1241–1243.
Mapes, R. W., D. S. Coleman, A. C. R. Nogueira, and T. B. Housh. 2004. “How Far Do Zircons Travel? Evaluating the Significance of Detrital Zircon Provenance Using the Modern Amazon River Fluvial System.” Geological Society of America, Rocky Mountain (56th Annual) and Cordilleran (100th Annual) Joint Meeting (May 3–5, 2004). Abstracts with Programs 36: 78.
Matthews, S. C., and J. W. Cowie. 1979. “Early Cambrian Transgression.” Journal of the Geological Society 136 (2): 133–135.
McLaurin, B. T., and R. J. Steel. 2007. “Architecture and Origin of an Amalgamated Fluvial Sheet Sand, Lower Castlegate Formation, Book Cliffs, Utah.” Sedimentary Geology 197 (3–4): 291–311.
Meert, J. G., and C. McA. Powell. 2001. “Assembly and Break-Up of Rodinia: Introduction to the Special Volume.” Precambrian Research 110 (1–4): 1–8.
Mints, M. V. 2007. “Paleoproterozoic Supercontinent: Origin and Evolution of Accretionary and Collisional Orogens Exemplified in Northern Cratons.” Geotectonics 41 (4): 257–280.
Mints, M. V. 2015. “Appendix II-4: North American Craton: Emergence and Evolution of the Paleoproterozoic Orogens.” In East European Craton: Early Precambrian History and 3D Models of Deep Crustal Structure. Edited by K. Condie and F. E. Harvey, 399–404. Geological Society of America Special Paper 510.
Mulder, J. A., K. E. Karlstrom, K. Fletcher, M. T. Heizler, J. M. Timmons, L. J. Crossey, G. E. Gehrels, and M. Pecha. 2017. “The Syn-Orogenic Sedimentary Record of the Grenville Orogeny in Southwest Laurentia.” Precambrian Research 294: 33–52.
Palmer, S. E., T. K. Kyser, and E. E. Hiatt. 2004. “Provenance of the Proterozoic Thelon Basin, Nunavut, Canada, from Detrital Zircon Geochronology and Detrital Quartz Oxygen Isotopes.” Precambrian Research 129 (1–2): 115–140.
Percival, J. A. 2004. “Orogenic Framework for the Superior Province: Dissection of the ‘Kenoran Orogeny’.” The LITHOPROBE Celebratory Conference: From Parameters to Processes—Revealing the Evolution of a Continent. Geological Survey of Canada.
Peters, S. E., and R. R. Gaines. 2012. “Formation of the ‘Great Unconformity’ as a Trigger for the Cambrian Explosion.” Nature 484 (7394): 363–366.
Piper, J. D. A. 2009. “Comment on ‘Assembly, Configuration, and Break-Up History of Rodinia: A Synthesis’ by Li et al. 2008 in Precambrian Research 160: 179–210.” Precambrian Research 174 (1–2): 200–207.
Piper, J. D. A. 2015. “The Precambrian Supercontinent Palaeopangaea: Two Billion Years of Quasi-Integrity and an Appraisal of Geological Evidence.” International Geology Review 57 (11–12): 1389–1417.
Pirajno, F. 1992. Hydrothermal Mineral Deposits: Principles and Fundamental Concepts for the Exploration Geologist. Berlin, Germany: Springer-Verlag.
Rainbird, R., P. Cawood, and G. Gehrels. 2012. “The Great Grenvillian Sedimentation Episode: Record of Supercontinent Rodinia’s Assembly.” In Tectonics of Sedimentary Basins: Recent Advances. 1st ed. Edited by C. Busby and A. Azor. Hoboken, New Jersey: Blackwell Publishing.
Rainbird, R. H., C. W. Jefferson, and G. M. Young. 1996. “The Early Neoproterozoic Sedimentary Succession B of Northwestern Laurentia: Correlations and Paleogeographic Significance.” Geological Society of America Bulletin 108 (4): 454–470.
Rainbird, R. H., V. J. McNicoll, R. J. Thériault, L. M. Heaman, J. G. Abbott, D. F. G. Long, and D. J. Thorkelson. 1997. “Pan-Continental River System Draining Grenville Orogen Recorded by U-Pb and Sm-Nd Geochronology of Neoproterozoic Quartzarenites and Mudrocks, Northwestern Canada.” Journal of Geology 105 (1): 1–17.
Rainbird, R. H., N. M. Rayner, T. Hadlari,, L. M. Heaman, A. Ielpi, E. C. Turner, and R. B. MacNaughton. 2017. “Zircon Provenance Data Record the Lateral Extent of Pancontinental, Early Neoproterozoic Rivers and Erosional Unroofing History of the Grenville Orogen. Geological Society of America Bulletin. doi.org/10.1130/B31695.1.
Rainbird, R. H., and G. M. Young. 2009. “Colossal Rivers, Massive Mountains and Supercontinents.” Earth 54 (4): 52–61.
Rino, S., Y. Kon, W. Sato, S. Maruyama, M. Santosh, and D. Zhao. 2008. “The Grenvillian and Pan-African Orogens: World’s Largest Orogenies Through Geologic Time, and Their Implications On the Origin of Superplume.” Gondwana Research 14 (1–2): 51–72.
Rivers, T. 2015. “Tectonic Setting and Evolution of the Grenville Orogen: An Assessment of Progress Over the Last 40 Years.” Geoscience Canada 42 (1): 77–124.
Saharia, M., P.-E. Kirstetter, H. Vergara, J. J. Gourley, and Y. Hong. 2017. “Characterization of Floods in the United States.” Journal of Hydrology 548: 524–535.
Schermerhorn, L. J. G. 1974. “Late Precambrian Mixtites: Glacial and/or NonGlacial?” American Journal of Science 274 (7): 673–824.
Sigler, R., and V. Wingerden. 1998. “Submarine Flow and Slide Deposits in the Kingston Peak Formation, Kingston Range, Mojave Desert, California: Evidence for Catastrophic Initiation of Noah’s Flood.” In Proceedings of the Fourth International Conference on Creationism. Edited by R. E. Walsh, 487–501. Pittsburgh, Pennsylvania: Creation Science Fellowship.
Sloss, L. L. 1964. “Tectonic Cycles of the North American Craton.” In Symposium on Cyclic Sedimentation. Edited by D. F. Merriam, 449–459. Kansas Geological Survey, Bulletin 169.
Snelling, A. A. 2009. Earth’s Catastrophic Past: Geology, Creation and the Flood. Dallas, Texas: Institute for Creation Research.
Spencer, C. J., and C. L. Kirkland 2016. “Visualizing the Sedimentary Response Through the Orogenic Cycle: A Multidimensional Scaling Approach.” Lithosphere 8 (1): 29–37.
Stern, R. J. 2005. “Evidence from Ophiolites, Blueschists, and Ultrahigh-Pressure Metamorphic Terranes That the Modern Episode of Subduction Tectonics Began in Neoproterozoic Time.” Geology 33 (7): 557–560.
Ten Bruggencate, J. 2001. Hawaii Land of Volcanoes. Mutual Publishing: Honolulu, Hawaii.
Timmons, J. M., M. K. Karlstrom, and C. Dehler. 1999. “Grand Canyon Supergroup Six Unconformities Make One ‘Great Unconformity’. A Record of Supercontinent Assembly and Disassembly.” Boatman’s Quarterly Review 12 (1): 28–32.
Timmons, J. M., K. E. Karlstrom, C. M. Dehler, J. W. Geissman, and M. T. Heizler. 2001. “Proterozoic Multistage (ca. 1.1 and 0.8 Ga) Extension Recorded in the Grand Canyon Supergroup and Establishment of Northwest- and North-Trending Tectonic Grains in the Southwestern United States.” Geological Society of America Bulletin 113 (2): 163–181.
Van Kranendonk, M. J., and C. L. Kirkland. 2013. “Orogenic Climax of Earth: The 1.2–1.1 Ga Grenvillian Superevent.” Geology 41 (7): 735–738.
Wingerden, C. V. 2003. “Initial Flood Deposits of the Western Northern American Cordillera: California, Utah and Idaho.” In Proceedings of the Fifth International Conference of Creationism. Edited by R. L. Ivey, Jr., 349–358. Pittsburgh, Pennsylvania: Creation Science Fellowship.
Yeo, G. M. 1981. “The Late Proterozoic Rapitan Glaciation in the Northern Cordillera.” In Proterozoic Basins of Canada. Edited by F. H. A. Campbell. Geological Survey of Canada, Paper 81-10: 25–46.
Yonkee, W. A., C. D. Dehler, P. K. Link, E. A. Balgord, J. A. Keeley, D. S. Hayes, M. L. Wells, C. M. Fanning, and S. M. Johnston. 2014. “Tectono-Stratigraphic Framework of Neoproterozoic to Cambrian Strata, West-Central U.S.: Protracted Rifting, Glaciation, and Evolution of the North American Cordilleran Margin.” Earth-Science Reviews 136: 59–95.
Young, G. M. 1978. “Proterozoic (<1.7 b.y.) Stratigraphy, Paleocurrents and Orogeny in North America.” Egyptian Journal of Geology 22: 45–64.
Young, G. M. 1995. “Are Neoproterozoic Glacial Deposits Preserved on the Margins of Laurentia Related to the Fragmentation of Two Supercontinents?” Geology 23 (2): 153–156.
Young, G. M. 2013. “Precambrian Supercontinents, Glaciations, Atmospheric Oxygenation, Metazoan Evolution and an Impact That May Have Changed the Second Half of Earth History.” Geoscience Frontiers 4 (3): 247–261.
Whitmeyer, S. J., and K. E. Karlstrom. 2007. “Tectonic Model For the Proterozoic Growth of North America.” Geosphere 3 (4): 220–259.
Zhao, G., P. A. Cawood, S. A. Wilde, and M. Sun. 2002. “Review of Global 2.1–1.8 Ga Orogens: Implications For a Pre- Rodinia Supercontinent.” Earth-Science Reviews 59 (1–4): 125–162.