Research conducted by Answers in Genesis staff scientists or sponsored by Answers in Genesis is funded solely by supporters’ donations.
Abstract
This introduction is the first of five papers on the philosophy of science, in which I discuss what science is perceived to be today, as well as some problems in science education. The philosophy of science involves the definition of what science is and the description of how science is done. Unfortunately, the state of education in the philosophy of science today is not good. Most treatments of the subject are mere introductions, leaving the impression that the scientific method is a sort of recipe that one may follow. There tends to be an inverse relationship between the depth at which science is taught and the amount of time spent instructing students on how to do science. Rather than being a formal process, science is a process of drawing inferences about how the world works. While science generally utilizes inductive reasoning, it also requires deductive reasoning. Some sources offer abductive reasoning as the foundation of science, but one can view abductive reasoning as a variation on the inductive method. The philosophy of science continues to change. There are trends in the attitude about science today that are hostile to theism. In the next paper, I will trace the development of science from the ancient Greeks through the Middle Ages. In the third paper, I will discuss the transition to modern science four centuries ago. In the fourth paper, I will describe the consequences of the scientific revolution of the sixteenth century and the transition to modern science a century ago. In the fifth paper, I will offer suggestions to a more biblical approach to science. Since I am an astronomer, my treatment of these topics naturally has emphasis on astronomy and physics.
Keywords: science, philosophy of science, scientific method, inductive reasoning, abductive reasoning
Introduction
What is science? Merriam-Webster Dictionary defines science as “knowledge about or study of the natural world based on facts learned through experiments and observation.” Collins English Dictionary says science “is the study of the nature and behavior of natural things and the knowledge that we obtain about them.” The Cambridge Dictionary says that science is “the careful study of the structure and behavior of the physical world, especially by watching, measuring, and doing experiments, and the development of theories to describe the results of these activities.” The Free Dictionary defines science as “the observation, identification, description, experimental investigation, and theoretical explanation of phenomenon.” Dictionary.com defines science as “systematic knowledge of the physical or material world gained through observation and experimentation.” According to Wikipedia, science “is a systematic enterprise that builds and organizes knowledge in the form of testable explanations and predictions about the world.” Encyclopedia Britannica defines science as “any system of knowledge that is concerned with the physical world and its phenomena and that entails unbiased observations and systematic experimentation.” The Science Council, a royally chartered organization in the United Kingdom, says “Science is the pursuit and application of knowledge and understanding of the natural and social world following a systematic methodology based on evidence.”
I would not disagree with any of these definitions of science. Though these definitions vary in the amount and types of details included, one can see common characteristics in them all. Some of these details get into the process of how science is done, which I shall defer for discussion in a later paper. Nor do these definitions address the two different ways that science is often practiced today, operational (experimental and observational) science and historical or origin science. I shall say more about this later. For the purposes of this study, I will adopt this definition of science: the study of the natural world using the five senses. Some may complain that this definition is too broad. However, I think a very broad definition is required, followed by detail as to how science is done. As a biblical creationist, it is important to emphasize that God created the natural world. This definition of science (as well as the other definitions) can lead one to realize that science has limitations. I will defer discussion of those limitations to later as well.
In my formal education to become a scientist, I was never required to take a course in the philosophy of science. In discussion with my scientific colleagues, none of them were required to take a course in the philosophy of science either. When we arrived at graduate school, the professors and the textbooks used in our classes seemed to have assumed we already knew what science is and how science is done. Research usually is a central component of graduate degrees in science. It is this research in which graduate students are expected to learn the intricacies of scientific research and hone their skills in properly conducting research.
So where are prospective scientists expected to have learned what science is and how science is done? Was it in those science classes required for completing undergraduate degrees prior to admittance to graduate school? As I recall those undergraduate science courses that I took many years ago, I don’t remember formal discussion of what science is and how science is done either in the classes or in the textbooks. Having graduated from Bob Jones University, a Christian university with a Science Department committed to recent creation, I vividly remember discussion about limitations of science, but that was in the context of the question of origins. That instruction was not so much concerned with what science is or how science is done, with the assumption seeming to be that students already knew what science was prior to going to university. I suspect that undergraduate science majors at most other universities did not even have the benefit of that sort of discussion. At universities, about the only science textbooks that include discussion of what science is and how science is done are those used in survey level courses for general education requirements.1 But not all those textbooks have such discussion, and many professors teaching those introductory classes for non-science majors elect not to cover that material in their lectures anyway.
Since undergraduate university professors often seem to assume their students already know what science is and how science is done, where are students expected to learn about the philosophy of science? The obvious answer is in high school science classes. Indeed, my experience with high school science curricula2 reveals that discussions of what science is and how science is done is commonly found in the first chapter of high school science class textbooks, particularly at the lower levels, such as physical science or general biology, that students often take as freshmen or sophomores. But how often do teachers stress this material? As one searches earlier in science education, such as in grade school, discussion of what science is and how science is done assumes a larger role in the curricula. Judging by the online resources that I have examined, teachers in these early grades are expected to put greater emphasis on what science is and how science is done than teachers in higher grade levels.
Over decades, I have been asked to judge many science fairs. I have found that participation rates and quality of science fair projects generally reach maximum in middle school. The peak of performance usually is around seventh grade. By the time students reach high school, there must not be much emphasis on science fairs because there seem to be relatively few high school science fair projects. And the quality of those projects often is below that of middle school projects. I suspect this is directly related to a diminished emphasis on the philosophy of science in the upper grades. With an apparent decrease in emphasis of philosophy of science at higher levels of science education, I conclude that there is an inverse relationship between the level at which science is taught and the amount of discussion of philosophy of science.
Education instills deeper understanding of subjects as students progress toward higher grades and beyond. For instance, where I grew up, state education standards mandated that students take American history in fifth, eighth, and eleventh grades. The reason for taking American history three times was to accommodate the increasing ability of young people to comprehend things as they mature. Fifth grade history had emphasis on facts—names, dates, places, and events. In eighth grade, additional facts were introduced, but now reasons behind those facts were emphasized more. It wasn’t so much the who, when, where, and what, but also the why of events of history. In eleventh grade history, the curriculum called for more in-depth understanding of the issues that shaped history. Eleventh grade students were judged more mature to comprehend these things than fifth graders or eighth graders.
A similar thing is true in math instruction. Young students first learn to count, then do operations with numbers. Once those basic skills are mastered, more complex concepts are introduced, such as fractions, decimals, figuring areas and volumes, and doing rate problems. Along the way, concepts of geometry and algebra are gradually inserted into the math curriculum. By high school, most students are deemed ready for algebra, with some students going on to take courses in geometry, trigonometry, precalculus, and perhaps even calculus.
The same sort of progression goes on in science education. Students begin with general science in grade school, then move on to life and physical science in middle school, followed by more specialized science classes in high school, such as biology, chemistry, and physics. Except that the instruction in the philosophy of science seems to fall away as students progress into more specialized sciences. Apparently, it is assumed that all students need to know about the philosophy of science can be instilled in grade school, or perhaps middle school. As a result, most people have at best a middle school understanding of the philosophy of science, what science is and how science is done. Underlying this trend is the belief that science is a very simple process, but nothing could be further from the truth.
What is this greatly simplified method of doing operational (experimental/observational) science? It generally begins with observing some phenomenon in nature, which causes the scientist to question why this phenomenon took place. Next, the scientist does some preliminary research on the subject, which eventually leads to forming a hypothesis, with the hypothesis being a cause-and-effect relationship between two things. It is at this point most basic discussions of the philosophy of science will define a hypothesis as an educated guess. To test the hypothesis, the scientist conducts a controlled experiment in which the scientist varies an independent variable (the cause) and looks for a change in a dependent variable (the effect). If the expected relationship between the two variables is found, then the scientist concludes that the hypothesis is confirmed. If not, then the scientist is expected to go back to an earlier step, perhaps modifying the hypothesis and repeating the process until satisfactory results are obtained.3
While the work that scientists do sometimes resembles this very simplified, cookbook approach, it often does not. For instance, physicists often measure the properties of substances, such as density, hardness, thermal and electrical conductivities, melting and boiling points, specific heats, and coefficients of expansion. The labs accompanying physics classes certainly have many exercises of this nature. None of this sort of research conforms to the pattern of testing hypotheses as described in basic descriptions of how science is done. However, being the study of some aspect of the natural world, it is part of science. Measuring properties of physical things (such as measuring the earth’s size, discussed in the next paper) is called metrology. Metrology is a very important part of science.
The very simplified approach to science is often called “the scientific method.” I don’t recall “the scientific method” being discussed much if at all when I was growing up, but that was more than a half century ago. Times have changed. I recently visited a first-grade classroom. A large poster on the bulletin board in the classroom had “the five steps of the scientific method.” When I was in elementary school, science was not part of the curriculum until fourth grade.
Here is what Cowles (2020, 1–2) had to say about “the scientific method”:
Scientists and historians do not always agree, but they do on this: there is no such thing as the scientific method, and there never was. And yet, ‘the scientific method’ is alive and well. The idea of a set of steps that justifies science’s authority has persisted in the face of constant denials of its existence. Why? Because ‘the scientific method’ is a myth—and myths are powerful things. How we talk about science, how we account for its origins and argue for its results, instils mythical authority in some claims and invalidates others.
Cowles went on to trace the mythological scientific method to John Dewey in 1910. It seems that no one did science prior to the twentieth century.
While the greatly simplified cookbook approach to doing science may help students develop some critical thinking skills and get them through basic primary and secondary science classes, it ultimately does not serve students well. As students mature, they ought to be taught more about what science is and how science is done rather than just this very basic, overly simplified cookbook approach to science. The inverse relationship between the emphasis on the philosophy of science and the depth at which science is taught has led to disastrous consequences.
What sort of consequences? I’ll give one example. Early in 2016, I learned of the rapidly growing flat-earth movement. Thankfully, the alarming rate at which the flat-earth movement was growing seemed to have slowed tremendously around 2019 (Faulkner 2021a). Recognizing the threat that the flat-earth movement presented, I began researching the flat-earth movement to provide refutation of the arguments flat-earthers make. This work has resulted in numerous articles on the Answers in Genesis website. These include blogs, web articles, In-Depth articles, and one Answers Research Journal article. Ultimately, I published a book (Faulkner 2019b) about the flat-earth movement. Since the publication of my book, I have continued research on the flat-earther movement. I have commented on how flat-earthers misunderstand the scientific method (Faulkner 2020a, 2020b, 2021b). Using many quotes about the scientific method, flat-earthers argue that science is done solely by testing hypotheses through conducting experiments where the experimenter manipulates an independent variable that in turn affects a dependent variable. Flat-earthers conclude that unless something conforms to this very narrow, rigid standard, then it is not science, resulting in the notion that most of what most people consider science is not science at all.
How did flat-earthers reach such a ridiculous conclusion? The numerous quotes that they use are mostly from the internet, but they are from reputable sources, such as various science departments at major universities, though many of the sources are meant for primary and secondary school use. Even when such quotes come from introductory university science classes, this underscores the inverse relationship between the depth of subject coverage and discussions of what science is. Flat-earthers do not realize that these quotations are very simplified versions of how science is done and hence are not meant to be complete and comprehensive treatments. Rather, these references promote a sort of cookbook approach to science that fosters a rigid formalism that is foreign to science. Furthermore, these descriptions of the scientific method often are discipline-specific, with a heavy focus on experiments, to the detriment of other sciences and an observational approach to science. In their ignorance, flat-earthers treat these quotes as exhaustive of how science is done. Consequently, in flat-earthers’ eyes, if something does not resemble a seventh-grade science fair project, then it is not science. The problem is that few discussions of how science is done are advanced beyond the primary or secondary education level. This sets people up to fail in properly understanding what science is and how science is done. It is ironic that the increased emphasis on science education in the United States that began more than six decades ago in response to the launch of Sputnik failed to include philosophy of science education because it has resulted in a public that does not understand science any more than it did before Sputnik.
The Christian and creation communities are not immune to this problem. Have there been any attempts to rectify this problem among Christians? There have been a few. Some writings directed at Christian audiences discuss some aspects of the philosophy of science, but they have addressed other issues. Examples are The Philosophy of Science and Belief in God (Clark 1964), The Soul of Science: Christian Faith and Natural Philosophy (Pearcey and Thaxton 1994), and Creation and Change: Genesis 1:2–2:4 in the Light of Changing Scientific Paradigms (Kelly 1997, 2017). Such treatments tend to focus more on the creation/evolution debate than on the philosophy of science itself (Clark’s book was more in response to scientists who think science has disproved God’s existence). Perhaps the best discussion of the philosophy of science in the more general sense by a Christian author is that of Ratzch (2000). However, Ratzch is not a recent creationist. Nor is Ratzch a scientist—he is a philosopher.
The other works I just mentioned were mostly authored by non-scientists (though co-author Thaxton is a scientist). This reflects a common practice in the twentieth century of non-scientists, often philosophers, rather than scientists, writing about the philosophy of science. An example of this is J.P. Moreland’s Christianity and the Nature of Science (Moreland 1989), who is a philosopher. This excellent book is a discussion of the history of science and its relationship to Christianity, though it is not a treatment of the philosophy of science per se. It is a bit of a surprise that Christian philosopher Francis Schaeffer did not write explicitly on the philosophy of science.
A few creation scientists have written some about the philosophy of science (e.g., Morris 1984; Morris and Parker 1987), but they have focused even more on the question of origins than the aforementioned authors. In a series of papers, Reed and his collaborators (Reed et al. 2004; Reed and Williams 2011, 2012; Reed and Klevberg 2014a, 2014b, 2015) focused on the assumption of naturalism in natural history (geology). While these papers touched on the nature and practice of science in general, they were focused on historical geology rather than science in general.
One notable exception to this lack of discussion of the philosophy of science in the creation literature is that of Brand (2006). More recently, Wise and Spivey (2015) discussed the philosophy of science in the creation literature, though this is only an abstract of an oral presentation. I will discuss what Brand, Wise, and Spivey said in the fifth and final paper in this series. Rinehart (2019) very briefly but succinctly made some good observations on the philosophy of science that ought to guide creation scientists. Given this general lack of prominence of discussion of the philosophy of science in the creation literature, I will discuss the philosophy of science in a broader sense in this and subsequent articles.
What is Science?
The word “science” comes from the Latin word for knowledge. Therefore, broadly speaking, science is knowledge, and this is what this word meant for a long time. This is the meaning of science in older English translations of 1 Timothy 6:20–21. With the shift in the meaning of the word science over the past two centuries, more recent translations have correctly translated this as knowledge. In 1 Timothy 6:20–21, the Apostle Paul was almost certainly referring to Gnosticism, a problem in the early church that exists even today (Faulkner 2019a). The English word “science” originally meant any systematic study of a subject that is not an art. What is the difference between a science and an art? Generally, an art is subjective, while a science is objective. An art usually expresses knowledge, often in a subjective representation, while a science is a system of acquiring knowledge. Examples of arts would include the study of various languages. Arts in this sense are different from the fine arts, such as music, painting, and sculpture.
To help understand this, consider how the disciplines within a university are organized. A university is divided into various colleges. For instance, the Christian university I graduated from had five colleges: Education, Religion, Business, Fine Arts, and Arts and Sciences. In terms of the number of majors included as well as enrollment, the College of Arts and Sciences usually is the largest college at a university. This is because the College of Arts and Sciences is a catch-all for programs that do not fit into other colleges. As a university freshman, I puzzled over why the College of Arts and Sciences did not include many of what I thought were arts (they were in the College of Fine Arts) and included many majors that I did not think of being either an art or a science (such as history). It was not until years later I came to understand the classical, original meaning of the terms art and science that I finally understood why the programs that were in the College of Arts and Sciences were there.
The meaning of the word science has changed over the past two centuries. What we call science today originally was called natural philosophy. For example, the founding document of physics as we know it is Sir Isaac Newton’s Philosophiae Naturalis Principia Mathematica, originally published in 1687. Translated into English, this title is Mathematical Principles of Natural Philosophy, which might more appropriately be translated as Mathematical Principles of Science today. As late as 1830, the astronomer John Herschel, son of the more famous astronomer William Herschel, published a treatise on the philosophy of science entitled A Preliminary Discourse on the Study of Natural Philosophy (Herschel 1830). Even as Herschel published his book, other scientists were advocating a new name for natural philosophy, “natural science.” Eventually, the modifier “natural” was dropped. As would be expected, it took time for this transition of usage to take place. Well into this shift in terminology, William Thomson (aka Lord Kelvin) and Peter Guthrie Tait published two related books with natural philosophy in their titles, Treatise on Natural Philosophy in 1867 and Elements of Natural Philosophy in 1872. By 1900, the older term “natural philosophy” had largely disappeared.
As previously stated, the definition of science I adopt is the study of the natural world using the five senses. This restricts science to the study of the natural world. Note that natural in this context is not in contrast to artificial, or manmade, things (as some flat-earthers seem to think). Rather, natural here means in contrast to the supernatural, or metaphysical. This places supernatural things, such as God’s existence, outside the purview of science. Many scientists today commit this error, thinking that science can address the question of God’s existence (this was the point of Clark [1964]). While we may not use science to study God, it is within the purview of science to make an inference about His existence. We may use our five senses to empirically see complexity and design in the natural world, design that implies the existence of a Creator. This is the essence of what the Apostle Paul stated in Romans 1:18–20, that the world around us proclaims that God exists and He is very powerful.
This definition also limits the study of the natural world to what we can explore with our senses, and by extension, the scientific instruments scientists employ. This distinction to include scientific instruments is particularly important to my field of expertise, astronomy. Since astronomical bodies are at such remote distances, we generally cannot touch, taste, smell, or hear the subjects of our research. We may see them, but many astronomical bodies are too faint for the eye alone to see. That is why we use telescopes. But many astronomical bodies are too faint for astronomers to directly see them even through the largest telescopes. Very long exposure photographs will produce images that astronomers can then see, but someone might object that the astronomers are not seeing those bodies but merely viewing photographs of those bodies. What about reception of electromagnetic waves outside the relatively narrow part of the visible spectrum, such as radio waves? We cannot see any of these other electromagnetic waves, so they mostly escape detection by our senses, which one might argue violates the definition of science that I have adopted.
These objections are pedantic. There are many things in the natural world that we cannot directly experience with our senses. For instance, no one has ever interacted with an electron using his five senses. Yet, there is compelling evidence that electrons are real, and we know many of the electron’s properties, even though we still don’t know exactly what an electron is. How have scientists determined these things? It has been through many carefully planned and executed experiments. Scientists build the apparatuses to test their models of electrons, and the scientists use their five senses to interact with and receive data from their apparatuses to reach conclusions. That is why I insist on the understanding that my definition includes the use of scientific equipment.
Why did I find it necessary to include mention of use of the five senses? Couldn’t I have simply defined science to be the study of the natural world? There are two reasons why I included the five senses (with the implied extension to use of scientific equipment) in the definition of science. Both reasons relate to the process whereby science is done. The first is more practical. There are some concepts about the natural world that scientists have developed that are beyond our ability to test. For instance, in physics, string theory has been popular for a few decades. But string theory makes no predictions that are testable at this time, and likely will not be testable for a long time, if ever. If an idea cannot be tested, can we say that it is science? Similarly, cosmic inflation has been widely accepted by cosmologists since the 1980s, but there are yet no testable predictions of inflation models. Similarly, most astronomers have accepted the Oort cloud as the source of long-period comets for more than a half century, but there is yet no evidence of its existence. Until any of these concepts can be tested, I must treat them as just-so stories and not scientific ideas. I’m not saying that none of them exist. I am merely saying that currently they are beyond the ability of any method of science to determine their reality. Just because a scientist expresses an opinion about the physical world, it doesn’t mean that his opinion is true or is science.
The second reason is even more profound because it gets to the heart of how science is done. Science is based upon empiricism, what can be demonstrated through observation and/or experiment.4 This naturally brings in the use of the five senses. Contrary to empiricism is rationalism, the belief that one can correctly reach conclusions about the nature of the world using deductive reasoning. With rationalism, the use of senses is not nearly as important as with empiricism. Whereas rationalism relies upon deductive reasoning, empiricism often relies upon inductive reasoning. Therefore, it is proper that I briefly review the differences between these two types of reasoning.
Deductive and Inductive Reasoning
Formal discussion of deductive reasoning goes back to the ancient Greeks. Deductive reasoning proceeds from the general to specific. If the premises and facts of an argument are true, and if the rules of deductive reasoning are properly applied, then the conclusion reached is true. The primary deductive rule of inference is called modus ponens or affirming the antecedent. Modus ponens begins with a first premise, a conditional statement of the form p→q. The first premise is followed by a second premise (or antecedent), p, of the conditional statement. The conclusion, q, is the consequent. Modus ponens can be written in symbolic form as
1. p→q
2. p
3. q
Here is a less abstract example:
1. All dogs are mammals.5
2. Spot is a dog.
3. Therefore, Spot is a mammal.
The first premise often is called the major premise, while the second premise is called the minor premise. They are followed by a conclusion. This three-step process is called a syllogism. Notice that the syllogism started with a general statement (about dogs) that was applied to a specific case (Spot) to reach a specific conclusion (about Spot). This is what I meant by deductive reasoning going from the general to the specific.
This very brief description of a syllogism is not meant to be a comprehensive discussion of deductive reasoning, because formal rules of deductive reasoning are quite involved; entire textbooks have been written on deductive reasoning. Rather, this was meant to illustrate the nature of deductive reasoning as arguing from a general proposition to a specific conclusion. This raises the question of how general statements come to be accepted as true in the first place. For instance, in my example above, mammals were part of the major premise. How is it that mammals came to be recognized as a distinct group of animals, or that dogs are part of that group? This is where inductive reasoning comes in. Inductive reasoning argues from specific cases to a general conclusion.
Consider taxonomy, more specifically the recognized categories of dogs and mammals. By examining many specific examples, people came to realize that animals have similarities yet have differences. Cattle, elephants, bears, and mice are obviously very different. Yet, these diverse animals have some common traits. They all are warm blooded and have fur, presumably to maintain body heat. They all give live birth and suckle their young (though there are few mammals that do not give live birth). This contrasts with other animals that lack some or all these characteristics. For instance, while eagles are warm blooded, they are covered with feathers rather than fur. Furthermore, eagles give birth via eggs and don’t suckle their young. Turtles and lizards don’t share any of the four characteristics of the first group of animals, but they do share the last two characteristics with eagles, they lay eggs and don’t suckle their young. Noting these similarities and differences, long ago people classed cattle, elephants, bears, and mice into a group of animals with similar characteristics that we call mammals. Eagles share their characteristics with many other creatures that we call birds. In like manner, turtles and lizards are included in a group of animals we call reptiles. Despite the differences between these three classes of animals, they do share some anatomical characteristics, such as having backbones and bilateral symmetry of forelimbs and hindlimbs. Therefore, we include mammals, birds, and reptiles into a higher grouping called vertebrates.
Even within classes of animals such as mammals, there are obvious differences. Upon additional inspection (using our five senses), we can further subdivide mammals. Based upon anatomical differences, such as bone structure, teeth, and digestive systems, cattle are included in a group of mammals called ungulates, bears are included with a group called carnivores, mice are included with rodents, and elephants are included in yet another group. Like bears, dogs are classified with carnivores, but clearly there are differences between bears and dogs. Even further grouping is done, establishing smaller groups of animals with shared characteristics. This painstaking process is empirically based. Through this process, one can establish general principles. These general principles can form the major premises for use in deductive reasoning, as in the example above. While the methodology in taxonomy is empirical, it does not make use of inductive reasoning. For that matter, taxonomy does not make use of the cookbook approach to science of forming a hypothesis and testing that hypothesis with an experiment, but it is still science.
The following example will illustrate a more typical use of inductive reasoning. Suppose that a person had never seen a cow but now sees a brown cow. If this person is inquisitive, he will note several characteristics that this cow has, such as gross anatomy. This sort of examination will give the person an idea of “cowness” so that he will be prepared to recognize another cow when he perchance sees it. The person may or may not think the brown color is significant, that brown is the color of all cows. Either way, suppose that the person sees a second cow. This cow won’t be identical to the first cow, but it probably will be similar enough to the first cow so that the person realizes it is a cow. Any subtle differences that do not contradict the concept of cowness will be realized as variations within cows, or the observer may have to modify his initial impression of what characteristics cows have. For instance, if the first cow had horns, then the observer might conclude that all cows have horns, but if the second cow did not have horns, this would force a change in that conclusion. If this cow also is brown, the person may begin to formulate a hypothesis that brown is the color of all cows. What if this person continues to find and examine a growing number of cows, all brown? Such a circumstance would eventually lead even the slowest thinker to consider the all-cows-are-brown hypothesis.
Once this hypothesis that all cows are brown is formulated, how can this person go about determining if his hypothesis is true? The only sure way to prove the hypothesis is to examine all cows. Given the large number of cows around the world, such a Herculean task is not possible. This is where the approach of inductive reasoning as used in science comes in. The budding cowologist sets out to test his hypothesis by seeking out and examining more cows. With each positive result, finding more cows that are brown, the hypothesis is confirmed. Notice that the hypothesis is confirmed, not proven. All that is necessary to disprove the hypothesis is to find a single non-brown cow, but until all cows are examined, the hypothesis cannot be proved, as most people understand the meaning of proof. We often get sloppy when discussing science, saying that something is proved when technically it has only been confirmed. Evolutionists are notorious for this, but some creationists do it also.
This simple example and the brief discussion of inductive reasoning is not intended as an exhaustive treatment of inductive reasoning. Like deductive reasoning, inductive reasoning is far more involved than what I presented here.
Notice that in the testing of the all-cows-are-brown hypothesis, no experiment was conducted, at least as many elementary discussions of “the scientific method” would advocate. Those basic descriptions of how science is done lay out a rigid process of formulating an experiment where the experimenter varies an independent variable that causes a change in a dependent variable. The emphasis on this approach is to test a cause-and-effect relationship between the independent variable (the cause) and the dependent variable (the effect). While science sometimes is conducted in this manner, this is not the only way that science can be done. In fact, this very limited approach to science is the exception to the broader way that science typically is done. Rather than saying that science frequently is done with inductive reasoning, it is better to say that science is done empirically, for much of science is difficult to categorize so rigidly as inductive reasoning is generally understood.
Furthermore, scientists also use deductive reasoning. One way that scientists use deductive reasoning is in designing tests of hypotheses. If a hypothesis is true, then there will be consequences of that hypothesis. Deductive reasoning is the method of reasoning what those consequences may be. Deductive reasoning is further used to devise tests (experiments or further observations) of the hypothesis. For example, a classic grade-school science fair project is to investigate what a plant needs to grow and thrive. Generally, the hypothesis developed is that a plant requires soil, water, and sunlight to grow properly. Using deductive reasoning, one concludes that providing some plants all three requirements will result in healthy plants, but denying one or more of the requirements to other plants will produce unhealthy or even dead plants. This interplay between inductive and deductive reasoning scientists use is called the hypothetico-deductive method
Another way that scientists use deductive reasoning is in answering questions posed to them or questions they bring up themselves to explain some phenomenon. To answer one of these questions, scientists will start from a few principles that they have confidence are true (general statements) and then proceed with deductive reasoning to reach an answer (specific cases). An example of this is combatting the common misconception that hot water freezes before cold water freezes. To begin to answer this question, one must first accept the understanding that freezing of any liquid requires that the liquid lose heat. Since hot water contains more heat than an equal amount of cold water, then for hot water to freeze it must lose more heat than the cold water must lose in order to freeze. Assuming that all other factors are the same (the two samples of water have the same mass, are in identical containers, and are placed in identical freezers), the hot water initially will lose heat at a faster rate than the cold water will. However, the initially hot water will eventually reach the temperature the cold water was initially. By then, the cold water has cooled to a lower temperature. Before the initially hot water can freeze, it must reach the new temperature of the initially cold water. But when the initially hotter water reaches the new, cooler temperature the initially cold water had, the cold water will be colder still. This process continues with the result that the temperature of the initially hot water never catches up with the temperature of the initially cold water. Since the cold water had a head start over the hot water, the cold water will reach the freezing point more quickly than the hot water. Therefore, the cold water will freeze before the hot water will.
Someone may object that this discussion here sounds like Zeno’s paradoxes (a subject I shall address in more detail in the next paper) and hence the reasoning used is invalid. Indeed, there is some similarity between this reasoning and Zeno’s paradoxes, but there is a significant difference. Zeno’s most famous paradox involves two runners, Achilles and a tortoise, that travel at constant rates. But the cooling rates of the two samples of water are not constant. Among other factors, the rates of cooling depend upon the difference in temperature between the two water samples and the presumed constant temperature of their environments. Since in Zeno’s paradox Achilles is running at a constant rate much faster than the constant rate of the tortoise, if given enough time, Achilles will eventually overtake the tortoise. Zeno argued the matter in fractions of the distance between Achilles and the tortoise so that Achilles had to exhaust an infinite series in order to catch up to the tortoise, something that Zeno deemed impossible. The resolution of Zeno’s paradox is that each successive decreasing fractional distance requires an equally decreasing fractional time so that in the limit Achilles easily covers the distance between himself and the tortoise. In the example of two samples of cooling water, the cooling rates do not asymptotically approach zero until well after freezing occurs.
Abductive Reasoning
In the late nineteenth century, the chemist and philosopher Charles Sanders Pierce muddied the waters by introducing the concept of abductive reasoning, a topic that continues to be discussed and debated today. The difference between abductive reasoning and inductive reasoning is very subtle, so in many respects abductive reasoning may be viewed as a variation of inductive reasoning. How are inductive reasoning and abductive reasoning similar? They both argue from specific examples to general statements. Unlike deductive reasoning, one can never be certain that a conclusion reached using either inductive or abductive reasoning is true. Rather, both inductive reasoning and abductive reasoning attempt to find the probable explanation for phenomena. Abductive reasoning is often described as reaching conclusions with incomplete information. But the same can be said of inductive reasoning (one can always collect more data), so how is abductive reasoning different from inductive reasoning? As I said, abductive reasoning is still much discussed and debated. I’m not sure I completely understand the difference between inductive and abductive reasoning. Let us examine a few sources as to the differences between inductive and abductive reasoning.
One source (Science Editor 2021) gave these three short descriptions of the three types of reasoning:
- Deduction is a general-to-specific form of reasoning that allows one to apply known truths to specific instances with a high degree of certainty.
- Induction is a specific-to-general form of reasoning that tries to generate broad rules that can be applied in many circumstances.
- Abduction is a specific-to-general form of reasoning that specifically looks at cause and effect, often for a particular example.
And later elaborated:
The difference between abductive reasoning and inductive reasoning is subtle, and not universally agreed upon. Both use evidence to determine what is likely—but not guaranteed—to be true. Abductive reasoning looks for cause-and-effect relationships, while induction seeks to determine general rules. Both work with the information that is available, which is usually incomplete. A criminal trial is an application of abductive reasoning. The jury must decide whether the prosecution or the defense provides the most likely explanation for all the evidence.
A medical diagnosis is another example of abductive reasoning. Given a patient’s symptoms and medical history, what diagnosis would best explain their situation? A diagnosis is essentially a hypothesis, which can often be tested by taking blood samples, ordering X-rays, trying medications, etc. It’s common for patient information to be incomplete or inaccurate in some ways, and for a diagnosis to not explain all symptoms.
According to another source (Anonymous n.d.),
The difference between abductive reasoning and inductive reasoning is a subtle one; both use evidence to form guesses that are likely, but not guaranteed, to be true. However, abductive reasoning looks for cause-and-effect relationships, while induction seeks to determine general rules.
DeMichele (2018) put it this way:
The core concepts to remember are: deductive reasoning deals with certainty and involves reasoning toward certain conclusions, inductive reasoning deals with probability and involves reasoning toward likely conclusions based on data, and abductive reasoning deals with guesswork, involves reasoning toward possible conclusions based on guesswork (a best guess), it is a type of reasoning that is used in formulating a hypothesis for further testing.
In other words, Abduction is forming a hypothesis, induction is like analyzing the data from testing a hypothesis, and deduction would be used in drawing certain logical conclusions from the data gathered. (Emphasis in the original)
This last source is also included in Table 1.
Deductive | Inductive | Abductive | |
---|---|---|---|
Major Premise | All Men are Mortal | Most Greeks Have Beards | Observation: That Man Has a Beard |
Minor Premise | Socrates is a Man | Socrates is a Greek | Known Fact: Most Greeks Have Beards |
Conclusion (Inference) | It is Certain that: Socrates is Mortal (this is logically certain given the premises; if all men are mortal, then Socrates being a man must be mortal. Here you can see that if a premise is false, deduction can produce false conclusions). | It is “likely” that: Socrates has a beard (given the premises, the conclusion can be assigned a likelihood; this argument isn’t very compelling, but to explain that quality of induction here would be a rabbit hole). | Perhaps: This Man is Greek (a hypothesis based on an observation and a known fact; we can gather inductive evidence to test this hypothesis, for example by gathering more information about the origin of the man). |
Let me summarize what these sources said is the difference between inductive and abductive reasoning. Abductive reasoning attempts to provide an explanation of data interpreted in terms of known facts. One example given was diagnosing a medical problem. A physician will evaluate the symptoms a patient has (data) considering what the physician knows about various diseases and disorders (known facts). The physician then reaches a conclusion about what the likely underlying problem is. Note that sometimes there may be more than one possible explanation, but physicians generally stick with what is more probable. For instance, a patient living in the United States may have some symptoms of a rare tropical disease not indigenous to the United States. Since it is highly unlikely that his patient has this rare disease, the physician will rule out that possibility in favor of a more likely one. Physicians use the slang term “zebra” for this practice. This term refers to an aphorism introduced by University of Maryland School of Medicine professor Theodore Woodward in the late 1940s. He cautioned his interns that when you hear hoofbeats behind you, don’t expect to see a zebra. Why? Because in Maryland horses are quite common but zebras are rare, or even nonexistent outside of zoos.
Another example was in a criminal trial, where the jury is expected to determine which of the two versions of what happened presented in the trial, the prosecution’s version or the defense’s version, is more probable. Technically, since under English Common Law the accused is presumed innocent until proven guilty beyond reasonable doubt, the burden of proof is upon the prosecution. Laying aside that objection, the jury is expected to apply the law of parsimony, often called Occam’s razor, named for William of Ockham, a fourteenth century English philosopher and theologian (I will discuss Occam’s razor more in the next paper). Occam’s razor dictates that when confronted with two explanations for a phenomenon, the simpler explanation is more likely correct. Abductive reasoning implicitly applies Occam’s razor.
Prior to a trial, the police investigate to determine what crime has been committed and who to charge with committing the crime. What sort of reasoning do the detectives use? The fictional sleuth Sherlock Holmes often famously claimed that he deduced certain things about a crime, suggesting that he was using deductive reasoning. But, as many people have correctly pointed out, Holmes generally did not use deductive reasoning. Rather, they insist, Holmes used inductive reasoning (admittedly, “I induce that...” doesn’t have the ring of “I deduce that....”). But did Holmes use inductive reasoning? Given the discussion here, it is more likely that Holmes used abductive reasoning. Detectives, both real and fictional, use clues (data) to reach the mostly likely conclusion about what happened. Therefore, criminal investigations involve abductive reasoning.
Allow me to give one more example of abductive reasoning. Auto mechanics frequently use abductive reasoning to diagnose problems with vehicles. The clues they consider may include unusual sounds, vibrations or smells, overall poor performance, or a simple failure to operate. With modern automobiles, it is often a dashboard warning light that brings the customer to a garage. An auto mechanic will read the code that triggered the alarm. The code reveals what system has failed, but the mechanic will not immediately know exactly what the problem is. Sometimes it is simply a matter of a faulty sensor; generally, a faulty sensor is easier and less expensive to replace than repairing the device the sensor monitors. In all cases, a mechanic will formulate the most likely hypothesis that will explain the problem with a vehicle.
Like the mechanic, the person considering the problem in the other examples formed the most likely hypothesis to explain the phenomenon under investigation. A physician’s diagnosis is the most likely hypothesis to explain the patient’s symptoms. A detective makes an arrest based upon the most likely hypothesis of what crime was committed and who committed it. What about the jury hearing the evidence presented in a trial? They are not asked to form a hypothesis. Rather, the jury is asked to determine which hypothesis is most likely. In this sense, the jury employs abductive reasoning, but not in the same way as the other three examples. The jury is asked to determine which of the hypotheses presented in the trial is more probable. Notice the key point, that abductive reasoning is used to form hypotheses, which is part of how science may be done.
So, what role, if any, does inductive reasoning play in these examples? Once one forms a hypothesis using abductive reasoning, then one can use deductive reasoning to develop tests of the hypothesis. For the physician, these tests are various procedures that the patient is subjected to, such as blood or urine sample analysis, a throat culture, an X-ray, CT scan, MRI scan, or biopsy. The physician will predict that if his patient is suffering from condition X, then when a particular test is performed, the outcome of the test will be Y. For the auto mechanic, the test may be removal or close inspection of the faulty system or part in a vehicle. Again, a testable prediction of the result of the test is made. In a criminal investigation, further physical examinations can be used to test the hypothesis developed by the investigators. However, in all these cases, there may not be a definitive test one can devise.
In scientific investigation, the same process plays out. A scientist observes a natural phenomenon. Based upon what he already knows and what he can learn about this phenomenon, the scientist forms a hypothesis, what he considers the most likely explanation for the phenomenon. This is abductive reasoning. Assuming that the hypothesis is true, the scientist uses deductive reasoning to develop a test of the hypothesis. This test takes the form of “if this hypothesis is true, then future observation, x, or experiment, y, will produce the result z.” The scientist then uses inductive reasoning to conduct observation, x, or perform experiment, y, and analyze the result of the observation or experiment. If the result is as predicted, then the scientist has some confidence that his hypothesis is correct. Note that the scientist cannot be certain of his conclusion because there may be other hypotheses that he has not yet considered that equally explain the observational or experimental results. However, the confidence that one has in a hypothesis is greater after testing it in this inductive reasoning manner. Therefore, while there is uncertainty in the conclusion one reaches in inductive and abductive reasoning, the level of confidence when using inductive reasoning generally is greater than the confidence when using abductive reasoning.
Again, I caution that this approach of developing and testing hypotheses is only a part of science. Science consists of much more than this.
Falsifiability
Since the middle of the twentieth century, the most significant figure in the philosophy of science has been Karl Popper. Popper was not a natural scientist (his doctorate was in psychology). Thus, Popper’s work was a clear break from prior works on the philosophy of science that had been written by natural scientists. This began a trend, for after Popper there have been many nonscientists who have written on the philosophy of science. While Popper said that he did not learn of Pierce’s work until after he had published his own work on the philosophy of science, Pierce’s teaching had a strong influence on Popper. It is not unusual for a person so influenced to not be aware of his influencers. For instance, many people influenced by Karl Marx never read Marx.
More than Pierce, Popper emphasized the uncertainty in the conclusions one reaches using inductive and/or abductive reasoning. Since other, yet unconsidered hypotheses may equally explain observational or experimental results, how can one be certain that the hypothesis one has successfully tested is indeed true? One cannot be certain, so the key to having better confidence in a scientific conclusion is to repeatedly test that conclusion. This is not a matter of merely repeating or performing the same or similar observations and experiments again and again. Rather, these are new tests done in an entirely different way. Popper’s point was that any scientific idea must at least have the potential of being disproved. It is this possibility of proving false an idea that makes it scientific.
If an idea by its very nature cannot be falsified, then it is dogma, a faith statement, or just an opinion, not science. Bertrand Russell illustrated this principle with an analogy. Russell said that if someone claimed there was a teapot orbiting the sun beyond the earth’s orbit that was too small and too distant for any telescope on earth to detect, then such a claim could not be disproved. Hence, no one need take such a claim seriously, and it certainly isn’t scientific.6 Russell had intended his teapot analogy to apply to the existence of God, with theists sometimes demanding that others prove that God does not exist. Russell insisted that God’s existence cannot be proved or disproved, so it is not a scientific question.7 However, Russell’s teapot analogy applies to any claim that cannot be tested.
In this sense, science can never prove anything to be true, at least as most people understand the word proof. The general understanding of proof is in the context of establishing something to be true, that is, not false. If there is always uncertainty in scientific conclusions, and if scientists ought to always look for ways to disprove our cherished ideas, then proof hardly seems an appropriate term for describing conclusions that scientists reach. Therefore, it has become quite common for scientists now to say that science can’t be used to prove anything. Perhaps that is a bit too harsh. Certainly, non-scientists seem to struggle with this concept. For instance, I have heard flat-earthers frequently mock this sentiment. Apparently, most flat-earthers think science can be used to definitively prove things (for example, that the earth is flat).
If science cannot be used to prove anything, then this can lead to despair. After all, if one embarks on a career in science, a lifetime study to find truth about how the world operates, only to eventually discover that one can never learn truth this way, it can be depressing. This realization can lead to a utilitarian view of science, that scientists are not concerned with truth but instead are concerned with what works.
Theories
To understand the world, scientists develop theories. What is a theory? In everyday usage, a theory is a guess, a hypothesis, an idea that may make sense to the theorizer, but hardly has been tested and may contradict known facts. This sentiment often is expressed dismissively as “that’s just a theory,” meaning that it is not true. This is a false dichotomy, as this is not what a scientific theory is at all. Many people think of a theory springing from a hypothesis or related hypotheses that have undergone rigorous testing and hence have abundant observational and experimental support, but this isn’t quite right. A scientific theory is a well-developed idea about some aspect of the world. An idea cannot be a theory unless it enjoys abundant supporting evidence. An excellent example of a theory is James Clerk Maxwell’s four equations of electricity and magnetism. These four equations describe very well all that is known about electrical and magnetic phenomena. Foundational to the theory is that charges and magnets produce fields that other charges and magnets interact with. What is a field? A field is an alteration of space. It is this alteration of space that telegraphs the presence of the initial charge or magnets that other charges or magnets respond to. In this manner, charges and magnets can affect other charges or magnets without coming into direct contact with one another. Gravity also can be understood in terms of field theory—a mass alters space in its vicinity that other masses respond to. Physicists have found field theory to be very useful.
Is Maxwellian theory of electromagnetism true? Some scientists may opine that it is true. In the Popperian view, we can never know. Right now, Maxwell’s field theory is the best description of electromagnetism we have, though in Popper’s view, there may be an alternate theory yet unknown that equally explains electromagnetic phenomena. Or a yet uncontemplated theory may explain electromagnetic phenomena even better. But until someone falsifies Maxwellian theory, not many physicists are interested in pursuing that possibility. Why would they, if there is no known crucial test that could decide between the old theory and the new theory? But the utilitarian viewpoint is that it doesn’t matter. All we need concern ourselves with is that Maxwell’s theory works very well. What more can scientists expect from a theory?
I don’t find this rather pessimistic utilitarian philosophy appealing, but I must admit that I regularly practice a tenet of it. I keep in my office a model of the celestial sphere (see fig. 1). This model has at its center a 3-inch diameter earth globe surrounded by a 12-inch diameter clear plastic sphere on which small white dots representing stars are painted. The sphere has lines representing coordinates we astronomers use in the sky, along with a line representing the ecliptic, the earth’s orbital plane. A small ball representing the sun can be moved along the ecliptic by means of turning a knob. A steel shaft representing the earth’s rotation axis extend out to the representation of the north and south celestial poles on the sphere. The sphere rests on a base with cardinal directions indicated on it. Attached to the base are two rings, one representing the horizon and the other the celestial meridian.
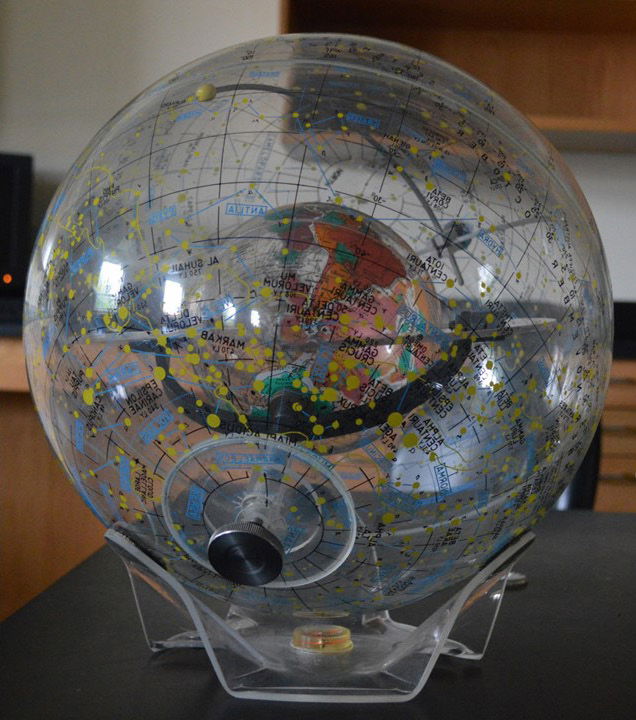
Fig. 1. A plastic model of the celestial sphere.
Is this model true? Hardly. Where do I begin with the problems with this model? It is geocentric, which I’m pretty sure isn’t true. The stars are all the same distance from earth—I am very certain the real stars are at various distances. I’m pretty sure that the stars are not attached to a hard, hollow sphere this way. And the stars’ distances are far out of scale with the earth’s size, as is the sun. I could go on, but you get the idea. So why do I keep such a flawed model on my desk? I use the celestial sphere model to illustrate many aspects of the sky, such as what stars are visible at a given time and date, as well as seasonal differences in daylight throughout the year, even though I know this representation isn’t true. You see, I am using a model not because it is true, but because it is useful.
How can such a flawed model do such a good job illustrating aspects of the sky? It turns out that given the great distances of astronomical bodies, our stereoscopic vision is of no help in discerning the distances of things above us. Therefore, the sky has a two-dimensional appearance, and as we move our gaze around, we mentally map this two-dimensional appearance onto a surface centered on us, making that surface a spherical shell. That is, while this model is not a good representation of what the world is truly like, it is a good representation of what we see. A planetarium does the same thing—the projection of small lights on the dome above us produces a good facsimile of what the night sky looks like.
As we shall see in the next paper, until about four centuries ago, the model on my desk was considered true by most people in the West. There are many other examples of theories in science formerly believed to be true but later were rejected but remain in use despite their flaws. For instance, heat was once thought to be a fluid called caloric. That theory ultimately was disproved and eventually was replaced with the kinetic theory of heat. But in thermodynamics we still talk about heat flow, as if heat is a fluid.
Explanatory Power vs. Predictive Power
A frequent claim today about scientific theories is that they must have both explanatory power and predictive power. This means that a good theory must explain what is already known and that it also makes predictions about future observations or experiments. This seems to conform to Pierce’s delineation between abductive and inductive reasoning, with abductive reasoning providing the explanatory power and inductive reasoning providing the predictive power. Scientists apply abductive reasoning to what we observe and already know about the world to develop hypotheses. We apply inductive reasoning to test those hypotheses. If a theory does a good job explaining what we already know and predicting outcomes that we don’t yet know, then it passes the test of being a good theory. Must a theory have equally strong explanatory and predictive power? That is a matter of judgement. I suspect that Pierce’s true agenda was to emphasize the explanatory power of some scientific theories while minimizing the necessity of their predictive power.
The nineteenth century saw the rise in naturalism in science, the assertion that the natural world is the only reality, or at least the only reality that matters. Though not explicitly stated, naturalism negates any place for a Creator. If there is no Creator, then the world and the things in the world came about through natural processes. The prime example of this thinking is biological evolution, that life began as simple, single-celled organisms that gradually developed into more complex living things. There is a progression found in the fossil record, with more complex organisms near the top and less developed organisms in lower strata. This broad fact would seem to comport with biological evolution. Thus, using abductive reasoning, naturalists of the nineteenth century began the shift in thinking that this progression of the fossil record is evidence for biological evolution.
In reality, abductive reasoning does no such thing. Abductive reasoning merely leads one to the possibility to consider biological evolution as an explanation for the fossil record; the fossil record is not evidence that biological evolution has occurred. How could one go about using inductive reasoning to provide evidence that biological evolution has occurred in the past? There is no observation one can make of a past process. Nor is there an experiment one can conduct to test a past process. It seems that the physical argument for biological evolution is based entirely upon the theory’s explanatory power with no regard to any predictive power. This was a profound shift in thinking in science.
Whether Pierce intended this shift, his introduction of abductive reasoning certainly helped facilitate the shift. This has led to a dichotomy in the way science is done. Creationists frequently note that science is used two ways today. One way is the traditional observational/experimental science that has been practiced for centuries. This study of the natural world as it now exists is sometimes called operational science. But the other way that science is carried out is origins/historical science. Origins/historical science is the study of what may have happened in the past. While observational/experimental science relies upon both abductive and inductive reasoning to some degree, origins/historical science relies entirely upon abductive reasoning.
Theories and Paradigms
The physicist Thomas Kuhn (1962) introduced the term “paradigm shift” into the English language. Most people had viewed the progress of scientific knowledge as being linear and continuous, but Kuhn argued this is false. In Kuhn’s view, scientific truth9 is determined by the consensus of the scientific community, with this consensus being the ruling scientific paradigm. Competing paradigms may exist alongside the ruling paradigm, but they typically are irreconcilable with the ruling paradigm and thus conflict with it. Consensus tends to enforce conformity, so a paradigm shift usually requires a crisis to exist before a new paradigm can be embraced by most scientists.
A good example of a paradigm shift is the revolution in physics in the early twentieth century (which I will discuss more fully in a subsequent paper). Introduced in the late seventeenth century, Newtonian, or classical, mechanics enjoyed two centuries of unparalleled success in describing the world. It seemed that ultimate truth about physics was understood and all that was required was working out the details. But in the late nineteenth century, experimental results began to accumulate that contradicted predictions of Newtonian physics. After a few decades of casting about, the foundation was laid for quantum mechanics and general relativity, the twin pillars of modern physics. There are many other examples of paradigm shifts in other sciences, such as geologists’ embrace of plate tectonics in the early 1960s, and the adoption of the heliocentric model over the geocentric model in the seventeenth century (which I shall discuss in a subsequent paper).
Kuhn’s thesis initially met with much criticism. It wasn’t that people disputed revolutions occurred in science. Rather, the critics disagreed with Kuhn’s assessment of how these revolutions occurred and why many scientists maintained the status quo even after the revolutions should have been over. If scientific truth is determined by a consensus of a scientific community, then there is a subjective element to that truth. But most scientists think of science being the pursuit of objective understanding of the world. If scientific truth is subjective, then how is it any different from other pursuits that are not primarily objective?
Kuhn’s vision of the progress of science resembles Hegel’s dialectic of thesis, antithesis, followed by synthesis. One scientific paradigm exists (thesis), but a new paradigm (antithesis) arises to compete with the ruling paradigm. A process of discussion, new experiments and observations, followed by more discussion leads to adoption of the new paradigm (synthesis). The revolution in physics a century ago is a good example of this. Newtonian mechanics was the thesis, but it was challenged by the antithesis of modern physics, quantum mechanics, and relativity (both special and general). But the triumph of modern physics did not lead to outright rejection of Newtonian mechanics. While quantum mechanics and relativity are thought to be more fundamentally true, in most applications in the macroscopic world there is no difference between the predictions of modern physics and classical physics, so in that sense physics today is a true synthesis of the thesis and antithesis.
This process is expected to continue. As I shall discuss in a subsequent paper, physicists await the emergence of a grand unified theory, the unification of the strong nuclear force with the electroweak force. This synthesis will not be the negation of the current understanding of the strong nuclear force and the electroweak force. Instead, it will be a synthesis of those two. Likewise, physicists expect that eventually a theory of everything will emerge in which gravity, the weakest of the fundamental forces of nature will be unified with the others. Will this be the end of the process? Current thinking says yes, but if the history of science is any indication, the answer is no.
However, some scientific revolutions do not lend themselves so much to Hegel’s dialectic in that the new paradigm may be so contradictory to the old paradigm that no synthesis is possible. Indeed, Hegel’s dialectic allows for contradictions to persist through the synthesis, but scientific ideas are not supposed to contain contradictions. None other than Popper (1944) harshly criticized Hegel in this regard. But some criticize modern physics for accepting apparent contradictions, such as light being both a wave and a particle and massless particles having equivalent mass.
Kuhn’s thesis ought to be very appealing to creationists. Scientists like to think of themselves as being objective, but the subjective component involved in forming and maintaining consensus reveals this is not the case. Furthermore, it is a pathway that can lead to seeing how important worldview is in reaching a consensus. Once a paradigm becomes firmly entrenched, it becomes part of one’s worldview. Underlying much of the scientific consensus today is the assumption of naturalism, though most scientists are not aware of it.
Natural Laws
There are many laws in science. Examples of laws in physics include Newton’s three laws of motion, Newton’s law of gravity, Coulomb’s law, and the four laws of thermodynamics. In biology there is the law of biogenesis. What is a scientific, or natural, law? The very term suggests something much beyond and of deeper significance than a theory. A law typically describes how the world works, a description that is universally true. How is a law established? That is, how does a principle discovered through scientific investigation come to be regarded as a law? There is no official process or committee to make that decision. A law becomes recognized as such by general usage, that is (with apologies to Kuhn), through consensus.
It is interesting that for the first two centuries of modern science, there were many principles that came to be viewed as laws. However, that practice did not continue into the twentieth century. I can’t think of a single scientific law that was recognized as such after the nineteenth century. It could be that nothing worthy of being termed a law has been learned since the nineteenth century, but it is more likely the result of changing attitudes.
It is no accident that modern science as we know it burst forth when and where it did, in Protestant Europe a century after the Reformation (Polkinghorne 1994; Ratzsch 2000). As we shall see in the next paper, Aristotle taught that the terrestrial and celestial worlds were different realms with different rules. In Aristotle’s view, the celestial world was perfect, but the earth was imperfect. This led to the conclusion that heavenly bodies were in perpetual motion on circular paths (what other shape is more perfect than a circle?). On the earth, rest was the normal state. For things to move, one must exert effort. This thinking dominated Europe for two millennia. As the Roman Catholic Church came to prominence in the Middle Ages, it embraced this and many other aspects of Aristotle and other Greek philosophers. While Aristotle tended to think of the separation of the earthly and heavenly realm more in physical terms, the Roman Catholic Church expanded this to the spiritual as well. Consequently, only heavenly work, the jobs of priests and kings, had worth in God’s eyes. All other work was venal, of no true value.
The Protestant Reformation tore down this barrier. All honest work is worthy of honor. If a farmer is called to be a farmer and he farms to the glory of God, then the farmer’s work is just as good in God’s sight as a priest’s or king’s work. This brought renewed vigor to labor, what we call the Protestant work ethic. But this barrier removal applied to the physical world as well. Though tainted by man’s sin, the creation is God’s handiwork and hence is worthy of honor as well. Thus, the study of God’s creation could be a God-honoring pursuit. This attitude is well expressed by a quote often credited to Johannes Kepler that he was merely thinking God’s thoughts after Him (Morris 1988, 12; Mulfinger and Orozco 2001, 22). Kepler did not explicitly state it this way, but it does capture the essence of Kepler’s sentiment when he wrote about the laws of nature that “God wanted us to...share in His own thoughts.” This attitude of praising God through their work was common among the founders of modern science.
This attitude is based upon the understanding that God upholds the creation moment by moment by the power of His word (Colossians 1:16–17; Hebrews 1:3). Since God is a God of order and decrees, then a good working hypothesis is that God sustains the world in an orderly and consistent manner so that one might expect to see patterns in the natural world. This was a revolutionary hypothesis because this concept was absent in the ancient Greek philosophy that came to dominate Europe. Ancient Greek deities were not much more than supermen and were thus incapable of creation, let alone sustaining creation.9 The Roman Catholic Church accommodated ancient Greek philosophy and even used it to interpret Scripture.10 Nor was this novel approach to the world possible in eastern philosophy, where chance and flux, the opposite of continuity, held sway. This new hypothesis was robust, as Newton and others discovered the unity of physical processes. This also brought about the understanding that the laws of physics that apply to heavenly bodies also apply to things on earth. This revolutionized and energized science.
Since God imposes this unity and order upon the world, if we discover principles that describe how this order plays out, then we can view these principles as God’s laws of how the world operates (this is where thinking God’s thoughts after Him comes in). That is, natural laws are expressions of what God, the Great Lawgiver, has ordained. This is the thinking that brought men to regard principles that describe actions in the natural world as laws. However, by the eighteenth century, atheism and agnosticism began to be tolerated and then became respectable. Soon, the assumption of naturalism, the denial of any supernatural agency, began to infect science. The vestiges of the belief in God as the author of nature remained in the nineteenth century, which is why some physical principles (such as the laws of thermodynamics) continued to be called laws. However, by the end of the nineteenth century, this thinking was largely deemed archaic. Unfortunately, this secularization of science is virtually complete today.
Trends in Science Today
As I previously discussed, the term science originally referred to any systematized study not considered an art. What we call science today originally was called natural philosophy. But in the 1830s, natural philosophy began to be recast as natural science, with the “natural” soon being dropped. What we now call the social sciences easily were sciences in old-fashioned use of the term, but do they fit in current use of the word science? Most natural scientists don’t think so. Indeed, many natural scientists dismiss the notion that the social sciences are sciences at all.
Many people in the social sciences view their work as being as much a science as the work of natural scientists. The foundation for this thinking was laid by Auguste Comte in the 1830s and 1840s in a philosophy that he called positivism. Positivism had a profound effect upon the natural sciences, which I shall discuss in Paper 4. While Comte was not the first to use the term sociology, he was the first to publish it. Why do many people in the social sciences insist that what they do is science? I think it is an attempt to fall under the mantle of certainty and respectability that society generally has given to the natural sciences. People in the social sciences wanted to replicate the wide success of the natural sciences. Eventually, social scientists attempted to quantify their work in much the way that many natural scientists had. Unfortunately, the social sciences usually engage in research projects that have many variables. Statistical approaches were developed to tweak the effect of the variables of interest from the maze of all the variable interactions.
These statistical approaches have become the stock in trade in medical research. Unlike the direct cause and effect relationships that often show up in the physical sciences, the many other variables in medical research often defy any direct cause and effect relationship. Only with statistical studies with large sample sizes can any sort of relationship be established. The classic example of this is the relationship between smoking and lung cancer. While there is a statistical relationship between the two, there are many individual cases that run counter to it. Most people who smoke do not develop lung cancer. Likewise, there are people who never smoke yet suffer lung cancer.
I am not denying that there is a real lung cancer risk when one smokes. I am saying that one must be careful in evaluating statistical studies. The initial health studies that used statistics to reach their conclusions, such as early ones linking smoking to lung cancer, showed a strong statistical correlation. Once that approach was established, health scientists began to reach conclusions based upon far less statistically significant studies. It is this slippery slope that many medical studies have descended into. There were studies that suggested coffee consumption was bad, but then there were studies that argued the opposite, while there were yet other studies that concluded that coffee drinking had beneficial effects. As I was writing an early draft of this paper, a study was published indicating that people at risk of heart disease ought not to daily take 81-mg aspirin tablets. This was after decades of studies that concluded daily intake of such low dose aspirin was beneficial in preventing heart disease. As an elderly person with a family history of heart disease, what am I to do? I fear that the many contradicting studies such as these have eroded public confidence in science.
Out of this new way to do science has emerged a sort of negative approach to proving a relationship between two things. Instead of providing evidence for a hypothesis, one articulates the null hypothesis, the negation of the hypothesis, and then one proceeds to disprove the null hypothesis. The rules of deductive reasoning dictate that if the negation of a proposition is false, then the proposition must be true. This method of proof by contradiction is well established in logic and mathematics, but it is a recent innovation in natural science. Imagine if Newton had set out to demonstrate the inverse square of distance relationship of gravity by assuming that gravity did not follow the inverse square law. That would strike most people as odd. Yet this approach is increasingly used in the natural sciences.
Throughout my career, I have witnessed these changes in the way science is done in my field of astronomy. Many major conclusions in astronomy, but especially cosmology, now are made on statistical grounds. This became apparent to me with the first claim of evidence of temperature fluctuations in the cosmic microwave background (CMB) in 1991. The researchers could not point to a single spot in the sky and say that it was definitely hotter or cooler than average. Yet, they had a high degree of statistical confidence that temperature fluctuations (that they didn’t see) were real.11 An even better example is the battle over the value of the Hubble constant that has been going on for two decades. The direct approach to the problem, measuring the redshifts and distances of galaxies with type Ia supernovae, yields a value of the Hubble constant around 73km/s/Mpc. On the other hand, highly statistical and model-dependent studies of the CMB produce a Hubble constant of around 67km/s/Mpc. The likely errors of these very different measurements of the Hubble constant do not permit both to be true. Perhaps I am a bit old fashioned, but I have always viewed statistics as providing the probable range of uncertainty that exists in the measurements we make, not in the conclusions we reach. Again, can anyone imagine Newton saying that he was convinced within five sigma that gravity follows an inverse square law of distance?
Finally, scientific consensus has become a
frequently used catchphrase today. This is particularly true in the discussion of anthropogenic climate change. It is also used against flat-earthers and biblical creationists. It seems that this catchphrase is used to silence anyone who dares question the scientific establishment. Scientists certainly can be cited as experts in their respective fields. However, merely asserting that scientists agree upon something without sufficient supporting evidence commits the informal fallacy of appeal to authority. After all, there are numerous examples from the history of science when the scientific consensus was wrong, which was a major part of Kuhn’s point. Not surprisingly, many of the people using this club probably would disagree with Kuhn.
Conclusion
What is science? And how is science done? There may not be any simple answers to these questions. In his concurring opinion in the 1964 Supreme Court case Jacobellis v. Ohio, Justice Stewart Potter was not able to define pornography, but he famously stated that “I know it when I see it.” Perhaps science and the scientific method are not easy to define either, but some people certainly know it when they see it.
When I was growing up in the 1960s, the library in my public school had copies of the How and Why Wonder Books series. Each volume was a book about some topic of science (later adding history), with each title being The How and Why Wonder Book of ______, with such titles as The How and Why Wonder Book of Dinosaurs and The How and Why Wonder Book of Stars. Looking back, I question the accuracy of these titles. Science usually reveals how things happen, but it doesn’t shed much light on why things happen. I used to tell my students that “science is pretty good at how, but not too keen on why.”
As I shall discuss in a future paper, Sir Isaac Newton published his theory of gravity in the Principia. Newton’s law of gravity is a masterpiece of physical science. It accurately described how gravity works. However, it did not address the question of the why of gravity at all. Newton had no idea what gravity is or why gravity exists. Nor do modern scientists. Modern scientists have only a modestly better understanding of gravity than Newton did. One aspect of gravity that baffled Newton was how gravity was able to reach out through seemingly empty space to act on bodies. All forces Newton was familiar with required direct contact, so gravity held out this mysterious property. When a friend wrote a letter to Newton enquiring why gravity operates, Newton famously replied to that question that he “framed no hypothesis.” Apparently, Newton had greater respect for the limitations of science than most scientists today. In subsequent papers, I will briefly trace the history of science and describe how people’s perception of science and how science is done has changed.
I will close with an amusing anecdote that illustrates the perils of drawing inferences. Many years ago, I heard an interview with a black man on National Public Radio who, like me, grew up in the 1960s. He related that one day he was in the home of a white friend when he happened to pass by the master bedroom with its door open. To his surprise, he saw that the bedroom had a double bed. He had seen master bedrooms of plenty of black households, and they always had a double bed, but the only master bedrooms of white families that he had seen were on television, and they always had twin beds. Therefore, as a child this man had reached the conclusion that white couples slept in twin beds while black couples slept in double beds.
This was a very reasonable, but false conclusion. What went wrong? There were no television shows depicting black families in the 1960s, and, like me, this young man wasn’t aware of the television networks’ moral code that existed at the time that was best met by depicting married couples sleeping in twin beds (at the time, I thought it was strange that couples on TV slept in twin beds). If this young man were aware of this additional information at the time, he likely would have formed a very different conclusion than the one he reached. This is a good lesson for all of us. We must be aware that no matter how well the inferences we draw in science match the data they are based upon, there always could be a better inference that equally explains the data we have in hand and potentially may explain data we don’t yet have.
References
Anonymous. n.d. https://courses.lumenlearning.com/boundless-psychology/chapter/reasoning-and-inference/.
Brand, Leonard R. 2006. “A Biblical Perspective on the Philosophy of Science.” Origins 59 (January 1): 6–42.
Clark, Gordon H. 1964. The Philosophy of Science and Belief in God. Nutley, New Jersey: Craig Press.
Cowles, Henry M. 2020. The Scientific Method: An Evolution of Thinking from Darwin to Dewey. Cambridge, Massachusetts: Harvard University Press.
DeMichele, Thomas. 2018. “Deductive, Inductive, and Abductive Reasoning Explained.” Fact/Myth July 10. http://factmyth.com/deductive-inductive-and-abductive-reasoning-explained/.
Faulkner, Danny R. 2019a. “Reflections on the Flat-Earth Movement.” Answers in Genesis, June 22. https://answersingenesis.org/astronomy/earth/reflections-flat-earth-movement/.
Faulkner, Danny R. 2019b. Falling Flat: A Refutation of Flat Earth Claims. Green Forest, Arkansas: New Leaf Press.
Faulkner, Danny R. 2020a. “The Scientific Method and the Flat Earth.” Answers in Genesis, January 31. https://answersingenesis.org/blogs/danny-faulkner/2020/01/31/scientific-method-and-flat-earth/.
Faulkner, Danny R. 2020b. “The Scientific Method and the Flat Earth II.” Answers in Genesis, October 28. https://answersingenesis.org/blogs/danny-faulkner/2020/10/28/the-scientific-method-and-the-flat-earth-ii/.
Faulkner, Danny R. 2021a. “Changes in the Flat-Earth Movement.” Answers in Genesis, February 12. https://answersingenesis.org/blogs/danny-faulkner/2021/02/12/changes-in-flat-earth-movement/.
Faulkner, Danny R. 2021b. “‘The Scientific Method’ and the Flat Earth III.” Answers in Genesis, October 8. https://answersingenesis.org/blogs/danny-faulkner/2021/10/08/the-scientific-method-and-the-flat-earth-iii/.
Herschel, John Frederick William. (1830) 1987. A Preliminary Discourse on the Study of Natural Philosophy. London, United Kingdom: Longman, Rees, Orme, Brown, and Green.
Kelly, Douglas F. (1997) 1999. Creation and Change: Genesis 1:2—2:4 in the Light of Changing Scientific Paradigms. Fearn, Scotland: Christian Focus Publications.
Kelly, Douglas F. 2017. Creation and Change: Genesis 1:2—2:4 in the Light of Changing Scientific Paradigms. 2nd ed. Fearn, Scotland: Christian Focus Publications.
Kuhn, Thomas S. 1962. The Structure of Scientific Revolutions. Chicago, Illinois: University of Chicago Press.
Moreland, J.P. 1989. Christianity and the Nature of Science: A Philosophical Investigation. Grand Rapids, Michigan: Baker.
Morris, Henry M. (editor). 1984. Scientific Creationism. 2nd ed. El Cajon, California: Creation-Life Publishers.
Morris, Henry M. 1988. Men of Science, Men of God: Great Scientists Who Believed the Bible. 2nd ed. El Cajon, California: Master Books.
Morris, Henry M. and Gary E. Parker. 1987. What is Creation Science? 2nd ed. El Cajon, California: Mater Books.
Mulfinger, George, and Julia Mulfinger Orozco. 2001. Christian Men of Science: Eleven Men Who Changed the World. Greenville, South Carolina: Ambassador Emerald International.
Pearcey, Nancy R., and Charles B. Thaxton. 1994. The Soul of Science: Christian Faith and Natural Philosophy. Wheaton, Illinois: Crossway Books.
Polkinghorne, John. 1994. Quarks, Chaos, and Christianity: Questions to Science and Religion. New York, New York: Crossroad.
Popper, Karl. (1944) 1966. The Open Society and Its Enemies. Vol. 2. 5th rev. ed. Princeton, New Jersey: Princeton University Press.
Ratzsch, Del. 2000. Science and Its Limits: The Natural Sciences in Christian Perspective. Downers Grove, Illinois: InterVarsity Press.
Reed, John K., and Peter Klevberg. 2014a. “Beyond ‘Origin’ and ‘Operation’ Science, Part I: Critique of OS2.” Creation Research Society Quarterly 50, no. 4 (Spring): 237–251.
Reed, John K., and Peter Klevberg. 2014b. “Beyond ‘Origin’ and ‘Operation’ Science, Part II: An Alternative.” Creation Research Society Quarterly 51, no. 1 (Summer): 31–39.
Reed, John K., and Peter Klevberg. 2015. “Battlegrounds of Natural History III: Historicism.” Creation Research Society Quarterly 51, no. 3 (Winter): 177–185.
Reed, John K., Peter Klevberg, Chris Bennett, Jerry Akridge, Carl R. Froede, Jr., and Thomas Lott. 2004. “Beyond Scientific Creationism.” Creation Research Society Quarterly 41, no. 3: 216–230.
Reed, John K., and Emmett L. Williams. 2011. “Battlegrounds of Natural History: Naturalism.” Creation Research Society Quarterly 48, no. 2 (Fall): 147–167.
Reed, John K., and Emmett L. Williams. 2012. “Battlegrounds of Natural History: Actualism. Creation Research Society Quarterly 49, no. 2 (Fall): 135–152.
Rinehart, L. 2019. “Philosophical Considerations on Biblical Creation Science.” Creation Research Society Quarterly 55, no. 3: 157.
Science Editor. 2021. “Your Guide to Deductive, Inductive, and Abductive Reasoning.” Servicescape, October 1.https://www.servicescape.com/blog/your-guide-to-deductive-inductive-and-abductive-reasoning.
Wise, K.P., and T. Spivey. 2015. “AIM Teleology and a Creationist Philosophy of Science.” Journal of Creation Theology and Science, Series B, Life Sciences 5: 7–8.