The views expressed in this paper are those of the writer(s) and are not necessarily those of the ARJ Editor or Answers in Genesis.
Abstract
One of several competing models, Hydroplate Theory (HPT) was proposed to simulate the initiation and ensuing tectonics of the Genesis Flood. HPT posits that wave-induced liquefaction sorted strata while subsequent tectonically-induced liquefaction resulted in the succession of faunal forms and the presence of cross-stratification throughout the stratigraphic record. Using the ubiquitous nature of ichnofossils throughout the stratigraphic record as a test point for HPT’s liquefaction submodel, this study questions HPT because liquefaction inherently erases ichnofossils by reorganizing the sedimentary fabric. As ichnofossils cannot be formed on the surface where they could be destroyed by liquefaction, Brown claims that ichnofossils were formed in subsurface liquefaction lenses between strata. This proposal not only results in conceptual contradictions and fails to preserve ichnofossils, but also cannot explain the order of terrestrial vertebrate tracks, making the liquefaction model questionable. Similarly, the association of tracks with coal seams challenges HPT’s liquefaction concept. Because of these challenges, HPT’s stratigraphic liquefaction model fails to coherently explain the nature of the stratigraphic record.
Keywords: Flood tectonics models, Hydroplate Theory, liquefaction, ichnofossils
Introduction
The nature of the stratigraphic record has truly captured the human imagination. Ever since seventeenth century savants recognized it as an incomplete account of geohistory, geologists worldwide have grappled with the intricacies of earth’s stratigraphy (Ager 1987, 1993; Reed 2005). It was from this burgeoning field of inquiry in the eighteenth century that deep time became entrenched in geology, yet further study has demonstrated naturalism’s repeated failures to explain the cataclysmic nature of earth’s stratigraphy (Reed 2010; Reed and Williams 2012). Instead, mounting data corroborates a global-scale catastrophe as recorded in Genesis (c.f., Clarey 2020; Oard and Reed 2017; Snelling 2009).
Based on the Genesis account, several models have been proposed to explain the tectonic and hydraulic processes active throughout the Genesis Flood. One such model is Hydroplate Theory (HPT), proposed in 1972 by Dr. Walt Brown and refined through nine editions of In the Beginning (Brown 2019). Based on specific initial conditions, Brown claims that the succession of fossil forms through earth’s stratigraphy was generated not by sequential inundations and deposition but as a function of buoyancy. Such sorting would have taken place during a phase of massive tectonically induced liquefaction in the Genesis Flood, thereby producing the continental-scale sequences of strata and forming what paleontologists refer to as faunal succession.
In particular, this paper will focus on HPT’s liquefaction-based stratigraphy and the distribution of biogenically produced sedimentary structures, termed trace fossils or ichnofossils, throughout the stratigraphic record. Including tracks, trails, and burrows, these traces document organismal interactions with the substrate, thereby recording numerous ethological and physiological details necessary for paleoenvironmental reconstruction (Bromley et al. 2007; Cowart and Froede 1994). Though claimed by some as support for HPT (Brown 2019; Nickel 2015), this paper will show how the trace fossil record refutes the basic tenets of a liquefaction-based stratigraphy.
Chronology of Hydroplate Theory
Based on a deductive approach, HPT proposes three initial conditions from which flows the resulting chronology (fig. 1):
- the created earth was encapsulated by a granite crust nearly 100 km thick.
- this crust was supported by downwarps in the granite crust creating pillars on a solid basalt mantle.
- The resulting void between the crust and solid mantle contained an interconnected shell of subterranean water averaging 1.6 km that sustained earth’s pre-Flood hydrologic system.
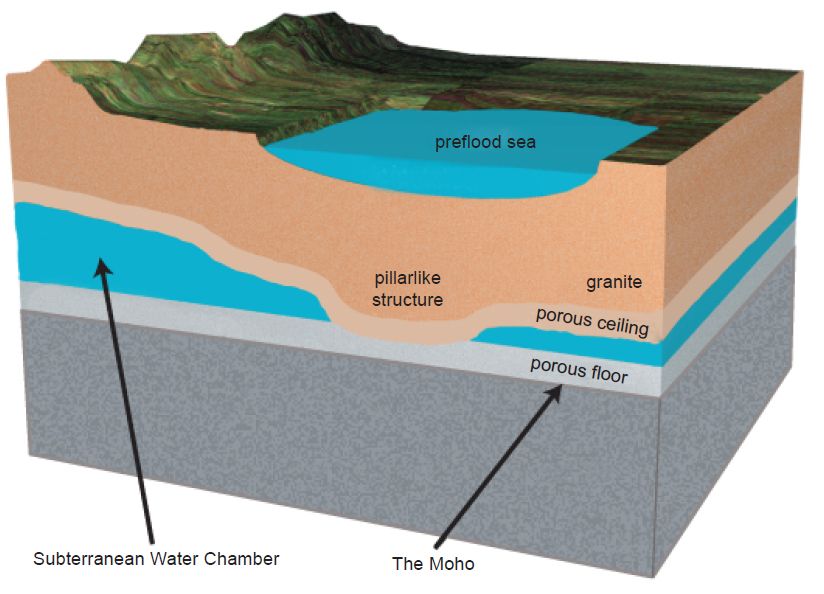
Fig. 1. The premise of Hydroplate Theory (HPT) relies on the validity of three primary assumptions, as shown in this depiction of HPT’s proposed crustal structure before the Genesis Flood. HPT purports the created earth was encapsulated by a granite crust nearly 100 km thick, which was supported by downwarps in the granite crust resting on a solid basalt mantle. The resulting void between the crust and solid mantle contained an interconnected shell of subterranean water averaging 1.6 km. Image from Brown (2019, 124, figure 42).
Within a decade of creation, lunar tidal pumping would have energized this subterranean water to the point the water reached supercriticality (Brown 2019, 477). This highly pressurized water dissolved minerals within the granite to produce a circulation network through the granite crust that fueled the light mists recorded in Genesis 2:6 (Brown 2019, 477). This is proposed to have continued as a stable system throughout the ensuing 1,600 years, though some critics have suggested a ticking time bomb scenario (Sarfati 2010).
The Genesis Flood would have commenced by crustal failure of the granitic crust creating a fracture that encircled the globe within a matter of hours (Brown 2019, 125). This released a torrent of highly pressurized subterranean water as the fountains of the great deep described in Genesis 7:11, initiating the inundation of the continents and the 40 days of intense precipitation (fig. 2a, table 1). Oscillatory vertical motion (“flutter”) along the retreating edge of crustal fragments (termed hydroplates) in response to repeated water hammers generated by escaping subterranean water would have stimulated tsunami-scale waves and associated wave-induced liquefaction across the continents, thereby sorting kilometers-thick sedimentary packages into strata of various thicknesses (fig. 3). Locations where rising water was entrapped between nascent strata or where vegetation mats impeded further ascension produced temporary water lenses or “liquefaction lenses,” preserving a disconformity. Though rising Floodwaters are believed to have assuaged the fountains of the great deep after the initial 40 days, sustained release of subterranean water provided a continual supply of granite lithics as the hydroplates were eroded.
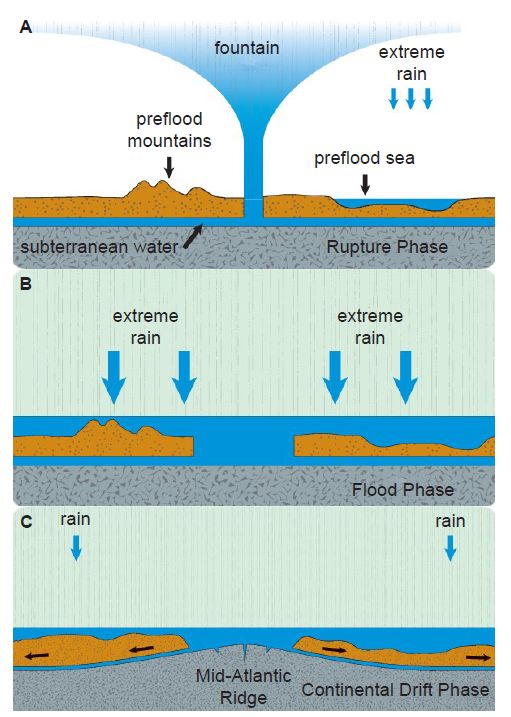
Fig. 2. HPT categorizes the Flood in three primary phases: (A) the Rupture Phase begun by crustal failure and the fountains of the great deep; (B) the Flooding Phase dominated by continued release of the subterranean water eroding the granite crustal fragments (hydroplates); and (C) the Continental Drift Phase initiated by the formation of the Mid-Atlantic Ridge. Collage produced from Brown (2019 figures. 44, 60, and 64, 125, 128, 131).
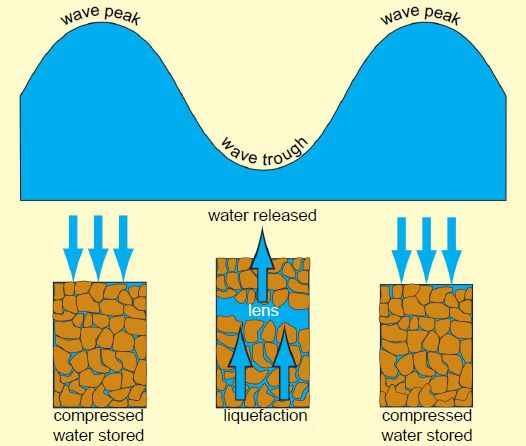
Fig. 3. Hydroplate Theory proposes two phases of liquefaction. The first phase, wave-induced liquefaction (pictured), would result from flutter at the edge of the granite hydroplates generating massive tsunamis. Each successive wave crest would force water into the sediment until the subsequent trough decreased pressure so that the escaping water carried smaller grain sizes from the larger surrounding particles, thereby sorting sediments by grain size. Repeated sorting would produce nascent strata that would be fine-tuned by later massive liquefaction during the Compression Event. Image from Brown (2019, 199, figure 33).
HPT Period | Geological Events |
---|---|
Creation | God creates Earth’s Pre-Flood structure (basalt basement rock overlain by inter-connected water channels and granitic crust). |
Pre-Flood period | Subterranean water becomes supercritical within a decade of Creation. |
The Flood: Rupture Phase | Crustal failure allows subterranean water to jet out (fountains of the great deep) and inundate the continents. Crack encircles Earth in two hours. |
The Flood: Flood Phase | Subterranean water continues to inundate the continents as Floodwater rises, causing wave-induced liquefaction. |
The Flood: Continental Drift Phase | Mid-Atlantic Ridge (MAR) buckles upward forming antipode Pacific Trenches. Continents slide away from the MAR. |
The Flood: Compression Event | Mountains form from the collisions and halting of the hydroplates during the Continental Drift Phase while massive liquefaction sorts the fossil record. |
Recovery Phase | Floodwater recedes from the continents, ending the Flood. Continents begin to stabilize as the Ice Age begins. Phase continues to the present. |
By the 150th day, erosion of the hydroplate margins had removed substantial pressure from the underlying subterranean chamber floor, causing it to up-buckle into the Mid-Atlantic Ridge (see Brown 2019, 130–131 and criticisms in Isaacs 2018, 2019). This upheaval allegedly caused the hydroplates to slide laterally from the rising Mid-Atlantic Ridge towards the concomitantly sinking Pacific Basin (fig. 2c) where the hydroplates eventually encountered resistances, deforming into today’s orogenic belts. Such rapid deceleration and compression of the hydroplates is believed to have induced massive liquefaction that rapidly sorted organisms into the present order based on their relative densities and buoyancies, purportedly explaining faunal succession. Concomitant drainage ushered in the post-Flood geologic and climactic recovery leading to modern times.
Brown’s Liquefaction-Based Stratigraphy
Resulting from seismic shaking or wave activity, liquefaction is the process whereby the behavior of a sedimentary package is transformed from that of a solid to that of a dense fluid. Most commonly occurring in low-cohesion coarse to fine sands, liquefaction is achieved when interstitial pore pressure is elevated to the point where the entrained water breaks the contacts between individual particles (Obermeier et al. 2002; Sassa 1996). Because the water is unable to bear the weight, however, the water is forced towards regions of lower pressure, often dissipating towards the surface along with loose sediment during what is called fluidization (Friedman, Sanders, and Kopaska-Merkel, 1992, 234). By reorganizing the sedimentary fabric, a variety of sedimentary features may be produced.
During fluidization, existent laminations may be warped to produce convolute bedding (Nichols 2009, 276), or the sedimentary package may be reorganized entirely into massive bedding (Boggs 2009, 84; Hildebrandt and Egenhoff 2007), thereby destroying any strata present previously. As readily seen in seismic hazard zones, fluidization during liquefaction may generate sand dykes leading to surficial sand volcanoes and sand blows formed as escaping water carries sand towards the ground level (Holzer 1998), though sand may conversely be injected into underlying sediment (Whitmore 2005; Whitmore and Strom 2010). Liquefaction can similarly generate a range of soft sediment deformation features if, for instance, the internal strength of a clay unit fails, allowing an overlying sand unit to settle as bulbous projections into the underlying clay unit (Miall 2016, 48–52). Regardless of which features are produced, however, liquefaction results in the reorganization of the sedimentary package to form newer, secondary features, thereby destroying artifacts of the original sedimentology. As such, liquefaction is inherently a destructive process by reorganizing the sedimentary fabric into new sedimentary features, erasing key information from the original stratigraphy.
Though readily observed in modern seismic zones or coastal sites, many such liquefaction and fluidization structures have been identified throughout the rock record. Soft sediment deformation is almost a ubiquitous aspect of the rock record (Miall 2016, 48–52). Possible liquefaction features associated with storm deposits have been proposed (Alfero et al. 2002), yet the most prominent example of liquefaction in the rock record is clastic dykes that may traverse hundreds of meters from their host sand unit (Whitmore and Strom 2010).
Besides these instances of liquefaction in the rock record, however, HPT proposes an alternative stratigraphy grounded upon the idea that the rock record is a direct result of continental-scale liquefaction. Brown (2019, 198) illustrates the HPT liquefaction concept:
The 10-foot-long metal beam pivoted like a teeter-totter from the top of the 4-legged stand. Suspended from each end of the beam was a 5-gallon container, one containing water and one containing a mixture of different sediments. A 10-foot-long pipe connected the mouths of the two containers.
Gently tipping the metal beam raised the water tank. Water flowed down through the pipe and up through the bed of mixed sediments in the other tank. If the flow velocity exceeded a very low threshold, the sediments swelled slightly as liquefaction began. Buried objects with the density of a dead animal or plant floated to the top of the tank. Once water started to overflow the sediment tank, the metal beam had to be tipped, so the water flowed back into the water tank. After repeating this cycle for 10 or 15 minutes, the mixture of sediments became visibly layered. The more cycles, the sharper the boundaries between sedimentary layers.
Though more precisely a demonstration of fluidization, this process becomes the foundation for the entire HPT liquefaction-based submodel. During the initial 150 days, wave-based liquefaction prevailed as repeated flutter along the leading edge of hydroplates generated tsunami scale waves across the hydroplates. Besides transporting newly eroded granite lithics, each successive wave crest and trough compressed and decompressed the surficial sediments. This cyclic action would sift sediments by grain size to a depth of 30 m below the water column (Brown 2011b, 382); further maturing of the sediments would produce bedding planes and disconformities wherever water temporarily accumulated in a lens beneath an impervious sedimentary unit.
Beginning on the 150th day, massive liquefaction dominated as the hydroplates decelerated during the Compression Event, forcing sediments to instantaneously dewater and liquefy en masse. Carcasses ascended through the liquified sediment based on their buoyancy to produce the order throughout the fossil record, while sediments were further divided by grain size and lensing concentrated plant mats at the base of cyclothems. Most sediments would be laterally continuous across the continent except when deceleration caused some sequences of strata to decouple from the adjacent strata, which became detached and slid laterally to form cross-beds as strata were tipped and beveled. As Brown (2019, 203) explains:
Likewise, each decelerating granite hydroplate acted on the bottom sedimentary layer riding on the hydroplate. Sedimentary layers, from bottom to top, acted in turn to decelerate the topmost layers. As each water-saturated layer decelerated, it was severely compressed—similar to suddenly squeezing a wet sponge. Sediments, forced into a denser packing arrangement, released water. Sedimentary particles were crushed or broken, so their fragments filled the spaces between particles, releasing even more water. The freed water, then forced up through the sediments, caused massive liquefaction. As the sedimentary layers decelerated and compressed, they became more and more fluid. Eventually, some layers were so fluid that slippage occurred above them, as in our [example] deck of cards. Below that level, extreme compression and liquefaction caused fossils to float up and collect at this watery level where sliding was taking place.
Brown claims that reorganization of the sedimentary fabric would have produced laterally continuous sheets of sediment across hydroplates, but beneath the primary slippage zone the strata were compressed and tipped diagonally:
As slippage began during the compression event, layers below the slippage plane continued to compress to the point where they tipped. The sliding sedimentary block above the slippage plane beveled off the still soft tops of the tipped layers. (Brown 2019, 203, 207)
Though Brown (2019, 195–210) provided illustrations of HPT anticipations, only Mitchell (2013) has applied HPT to a regional context. However, HPT remained merely an overarching paradigm, leaving the specific expectations and resulting application of HPT’s liquefaction model poorly developed. Even so, several predictions may be teased from the preceding chronology.
First, stratification results from two physically and temporally independent liquefaction mechanisms, both believed to produced laterally continuous strata. As discussed above, wave-induced liquefaction prevailed throughout the early Flood, but sorting would gradually become inconsequential once sediments were buried by more than 30 m. Though strata would be relatively well sorted by this initial process, subsequent massive liquefaction during the Compression Event would further stratify sediments en masse as the order seen within the fossil record was established. This final stage of liquefaction generated another phase of lensing that finalized the stratigraphic record as cementation commenced. Thus, the current rock record would be largely the result of massive liquefaction fine-tuning nascent strata formed by earlier wave-induced liquefaction.
Second, the fossil record is primarily a function of buoyancy. Deposition of organisms would be largely random because the fountains of the great deep were initiated near pre-Flood mountainous regions, leaving no sequential inundation of ecosystems. Similarly, wave-induced liquefaction simply stratified sediments.
Third, the stratigraphic position of sediments should record those processes active during their deposition. As noted in an analysis in Isaacs (2022):
First, sediments should be found near the stratigraphic level at which they were initially deposited. Brown said the fossil succession found within the stratigraphic record was produced by the relative buoyancy of animal carcasses, but sediment grains would have densities closer to each other than to carcasses. Therefore, grains would not rise to the surface during liquefaction but instead would be concentrated near the level where they were deposited, creating graded deposits and cyclothems as proposed by Brown. Thus, the presence or absence of a substance (e.g. lithic or mineral) in the stratigraphic record should reflect the processes active at the time a cyclothem or sequence was being deposited and initially sorted.
As such, only organismal remains would undergo appreciable vertical change. In contrast, the position of banded iron formations, volcaniclastics, and limestone beds should not have changed appreciably during liquefaction, thus leaving a somewhat event-based stratigraphic record. This, however, is in contrast to features in the sedimentary fabric such as soft-sediment deformation and clastic dykes which must occur during the closing stages of massive liquefaction.
Ichnofossils: A Vibrant Dataset for Diluvial Geologists
Ichnofossils are the remains of an organism’s interaction with the substrate preserved as biogenic sedimentary structures (fig. 4). These sedimentary features, also known as ichnites or trace fossils, may include burrows, tracks, and trails, which record important ethological and physiological characteristics of the producer (Bromley et al. 2007; Cowart and Froede 1994; Martin 2009). Because they are preserved strictly as a feature of the host fabric (most often sand), ichnofossils are primarily autochthonous and only rarely transported (Buatois and Mángano 2011, 15; Seilacher 1967). As such, ichnofossils may record specific details of the organism’s response to environmental conditions at the time of deposition. Because of these factors, many ichnofossils are strongly facies-controlled based on the composition of the substrate and other conditions. Certain ichnofossils, particularly Skolithos, may serve as geopetal devices recording the original orientation of the stratum at the time of the trace’s formation. The relative abundance of ichnofossils throughout the entirety of the stratigraphical record has made certain ichnofossils useful in biostratigraphy (Mángano and Buatois 2017; Mángano, Buatois, and MacNaughton 2012; MacNaughton 2007).
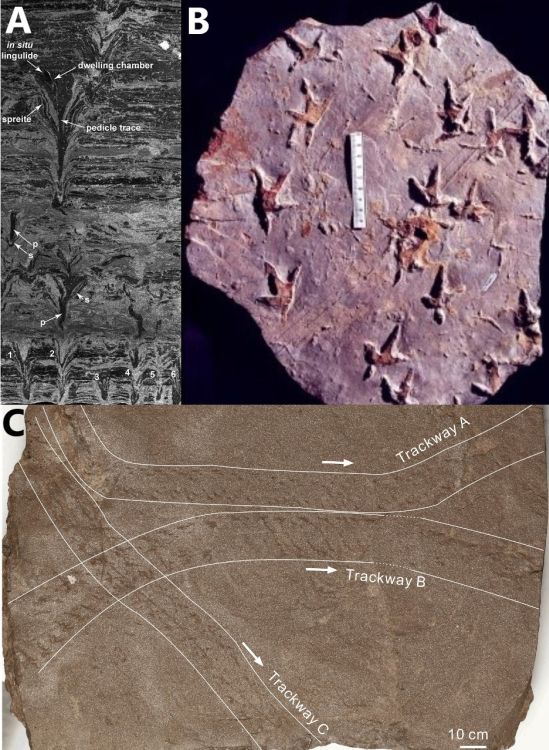
Fig. 4. Formed from organism-substrate interactions, ichnofossils or trace fossils record a host of physiological and ethological details of the producer in the sedimentary fabric in the form of trackways, burrows, and other biogenic sedimentary structures. A. Burrows formed by Triassic Lingulide brachiopods bioturbating very fine sands (Zonneveld and Pemberton 2003, figure 5a). B. Negative relief slab of Mesozoic bird tracks (Lockley et al. 2009, figure 7). C. Several trackways formed by Triassic Horseshoe Crabs (Shu et al. 2018, figure 12c).
Because of the information recorded by ichnofossils, ichnological datasets have become important for constraining both local and regional Flood models (see Austin et al. 1994; Froede 2010; Froede, Akridge, and Reed 2014; Woodmorappe 2006). In his chapter on liquefaction, Brown defines the meaning of ichnofossils within the constraints of Hydroplate Theory’s liquefaction model:
During the early weeks of the flood, flutter amplitudes were large enough for the crust to rise repeatedly, but slowly, out of the flood waters . . . Frightened animals—and sometimes dinosaurs—scampered uphill onto the rising land, each leaving footprints. Minutes later, the crust again submerged, allowing sediments falling through the thick muddy waters to blanket and protect the prints while the rising water swept the animals’ bodies away. Other perishable prints—called trace fossils—were made in the same way . . . . (Brown 2019, 200)
He further postulates that, “Sometimes, dinosaur prints from the previous upward flutter minutes earlier were sandwiched between layers that never experienced liquefaction again” (Brown 2019, 200).
Liquefaction: A Destructive Process
Although HPT relies on liquefaction to produce strata and the global succession of fossils, liquefaction inherently destroys ichnofossils as artifacts of the host sedimentary fabric. Surface features, such as tracks, are easily destroyed by the reorganization of the sedimentary fabric during processes as simple as erosion, while shallow burrows can be obliterated merely from the action along a dynamic shoreline. Because an ichnofossil is merely an impression on the surface of or a void within the substrate, reorganization of the sedimentary fabric during liquefaction would destroy trace fossils. Moreover, surficial trace fossils such as tracks and trails progress along bedding planes while burrows commonly bioturbate the strata, both of which would require strata-producing liquefaction prior to ichnofossil formation. These traces are almost invariably overlain by well-defined strata, whose very existence would indicate continued phases of deposition and liquefaction subsequent to the original production of the ichnofossils, as propounded by the wave-induced liquation concept (Brown 2019).
This is well illustrated by sauropod tracks at Dinosaur Ridge in Colorado. As seen in fig. 5, strata are clearly visible both overlying and underlying the tracks, but the strata below the track have been deformed in an “underprint”; the presence of non-deformed strata overlying the track but deformed strata below the track indicates that strata had already been formed before deformation by the sauropod and was followed by further stratification after the track was produced. How could liquefaction preserve such a track while producing strata mere centimeters above the track? Indeed, if wave-induced liquefaction is purported to have extended to depths of thirty meters, how could one expect tracks to survive within a few centimeters of the surface? Brown (2019, 200) attempts to circumnavigate this challenge by claiming that, “Sometimes, dinosaur prints from the previous upward flutter minutes earlier were sandwiched between layers that never experienced liquefaction again.” This claim contradicts Brown’s entire liquefaction model, which is predicated on the assumption that wave-induced liquefaction and later massive liquefaction during the Compression Event produced earth’s strata.
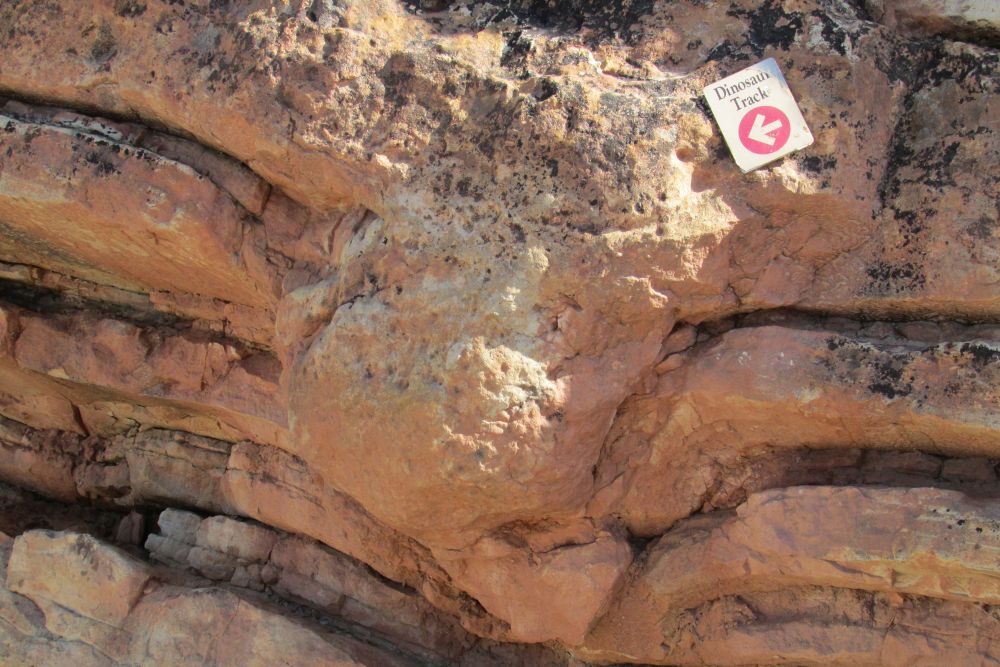
Fig. 5. A sauropod track popularly known as a “Brontosaur Bulge” from Dinosaur Ridge in Colorado. Observe that the track deforms the underlying strata and is overlain by further stratified sediment. Cumulatively, this indicates that sediment was stratified before and after the production of the tracks, but how could liquefaction preserve such a track without destroying it in the process of producing strata mere centimeters above? Photograph by Chris Light in the public domain under the CC BY-SA 4.0 license. https://commons.wikimedia.org/wiki/File:Bronto_Bulges_2017-09-30_1638.jpg.
In many cases, ichnofossils were formed either along the surface or within a meter of the substrate/ surface interface, yet liquefaction is proposed to continue to depths exceeding 30 m (Brown 2011b, 382), while percolating water from subsurface lensing would continue to damage ichnofossils. Thus, no ichnofossils would be exempt from repeated phases of destructive liquefaction regardless of original burial depth. Although sand is the most susceptible to liquefaction (Obermeier et al. 2002), it is the most common host of ichnofossils in the stratigraphic record (Buatois and Mángano 2011, 15; Seilacher 1967).
Liquefaction Lenses: A Flawed Solution
Because of the impossibility of forming ichnofossils at the surface, HPT is forced to find another means to preserve ichnofossils while producing strata. In an exchange with creation educator Ian Juby, Brown (2011a) explained that ichnofossils could only be preserved after the final stage of liquefaction in an area. This would occur following the massive liquefaction during the Compression Event (see overview above). Besides producing faunal succession, this is proposed to create another cycle of liquefaction lensing. As water is impeded from ascending into the overlying strata, it would form liquefaction or water lenses between nascent strata as the water percolated upward. This would render cavities perhaps a meter thick that would allow for the production of ichnofossils to be preserved after the final liquefaction lens closed. Brown (2011a, 338) illustrates this proposition using the tracks of the Coconino Sandstone at the Grand Canyon, saying:
The last and largest liquefaction cycle was during the powerful compression event—after the flood phase . . . Therefore, temporarily buried amphibians [that produced the tracks of the Coconino Sandstone] during the early weeks of the Flood could have been released within a lens of water for periods of a minute or so. They would have scampered uphill (as almost all footprints show), in a direction that the animals thought might free them permanently. If they were still alive during the compression event, they could have been released for even longer and been able to make footprints with toes pointing in quite different directions from their actual movement. . . . After the last liquefaction cycle at a location, the footprints made minutes before by the scampering amphibians would be preserved as the roof of the liquefaction lens settled onto the floor of the lens.
However, Brown does not demonstrate how these “liquefaction lenses” may be produced; instead, they are integrated into the HPT liquefaction submodel conceptually without experimental support. This is troubling, particularly because liquefaction throughout a sedimentary package commonly destroys bedding by homogenizing the sediment rather than producing distinct layering (Boggs 2009, 84; Hildebrandt and Egenhoff 2007). Such laminations are instead produced when the sand-laden water ascends through the sediment and deposits its load along the surface as sand volcanoes and sand blows (Holzer 1998). As such, these liquefaction lenses, if produced, would be choked with sand, a dense medium impeding movement of any organisms that may find themselves within the liquefaction lens.
Even if some claim that this could potentially explain trace fossils from invertebrate and non-terrestrial vertebrate producers, this example fails to explain those traces formed by terrestrial vertebrates such as reptiles and mammals, which could not survive in a liquefaction lens long enough to form ichnofossils capable of preservation. Indeed, even this very case contradicts HPT postulates. First, Brown states that the tracks were formed as their amphibian producers climbed the cross-beds in search of safety, yet Brown later contradicts himself by stating that the cross-beds formed after the tracks:
some [of the producers] might have been pulverized by the friction between the thin, but compact, sliding layers [that became cross-beds] . . . Therefore, footprints might have been preserved, although soft bodies were pulverized. (Brown 2011c, 431)
Even so, this also is impossible, as the tracks indicate that the amphibians ascended along a slope and thus suggest cross-beds were already present, but liquefaction, when it generates lamination, generally forms horizontal bedding (Friedman, Sanders, and Kopaska-Merkel 1992, 234). The cross-beds are therefore required to be present contemporaneous to the amphibians, but this would require HPT to assert that lensing happened intermediate of individual cross-beds to allow the amphibians to propel themselves across the tilted cross-beds. This lensing would not only destroy the cross-stratification but would also form horizontal lenses transecting those cross-beds because of the permeability of the coarse sand, falsifying the entire HPT narrative for these tracks.
Geopetal Devices in Cross-Beds
Liquefaction-based decoupling of cross-stratification has been highlighted as a strongpoint of the HPT liquefaction submodel (Brown 2019; Nickel 2015). HPT’s competitor of bedform stratification is claimed to suffer from an apparent lack of source materials along a properly elevated gradient (Brown 2011b, 383), yet Brown’s proposal itself suffers from a space problem. After all, voluminous quantities of sand must be removed during the beveling of laterally continuous sandstone beds from many kilometers in extent to mere meters in length (fig. 6). This is compounded by the presence of geopetal devices, which denote the original orientation of strata. As seen in fig. 7, Skolithos is a vertical burrow that is often found in cross-stratified coarse sands (Pemberton, MacEachem, and Frey 1992). If cross-beds had formed from decoupled and tipped strata, the Skolithos burrows should be parallel to the beds and offset along bedding planes, yet the burrows remain vertical regardless of the stratification orientation. Even lensing between individual cross-beds, as proposed for the tetrapod tracks in the Coconino Sandstone, fails because the sandstones would often be too porous to cause lensing. Rather than a strongpoint for HPT’s liquefaction submodel, cross-stratification exposes HPT’s weaknesses: it suffers from an inability to explain geopetal burrows.
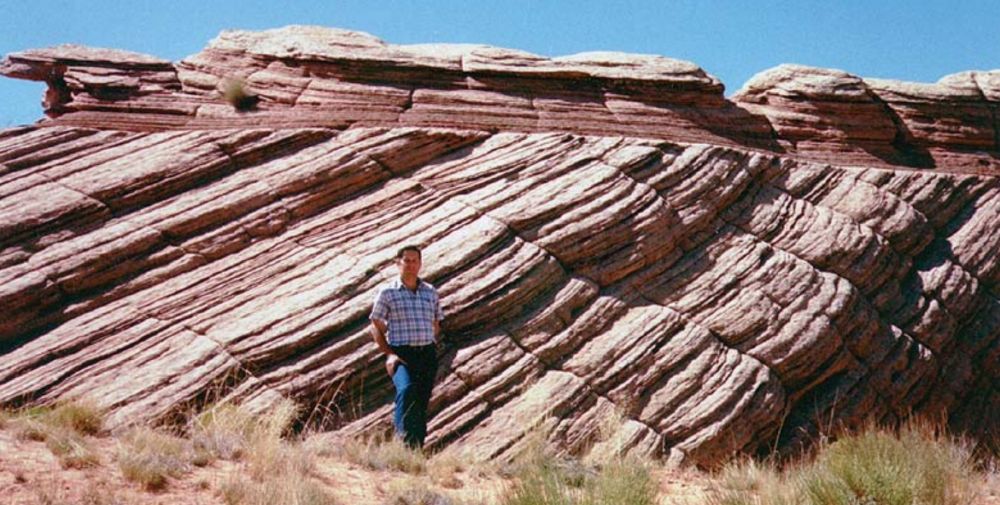
Fig. 6. HPT’s liquefaction submodel explains cross-beds as originally horizontal strata that were tipped and beveled as the continents decelerated during the Compression Event. As illustration, Brown (2019, 208, figure 115) describes the lower cross-beds as decoupling from the overlying strata, tipping diagonally, and becoming truncated as the overlying beds slid across. However, this suggests that cross-beds, once horizontal strata extending many kilometers, are mere vestiges of much more voluminous strata, yet the removal of this excess sand remains unexplained because neither clastic dykes nor the erosion of the canyon landscape much later can be invoked to remove the sand. This leaves HPT with a space problem challenging its notion of a liquefaction-based stratigraphy.
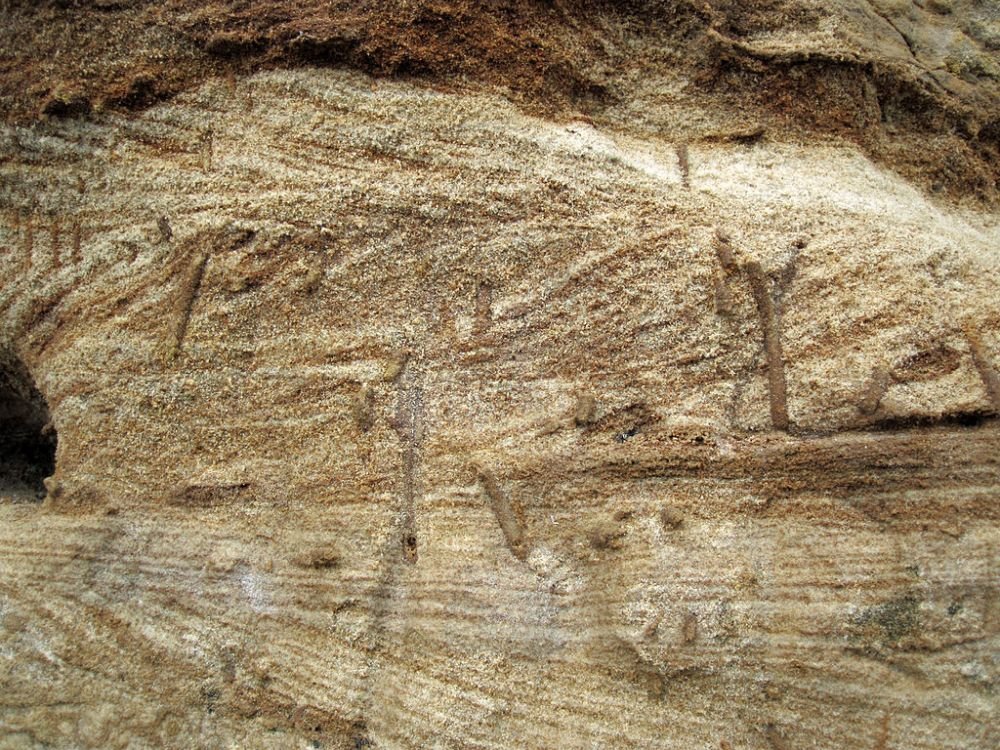
Fig. 7. As seen from the Upper Cambrian Mazomanie Formation in Minnesota, USA, Skolithos is a common vertical burrow in the ichnological record. Strongly facies controlled, Skolithos burrows are geopetal devices by indicating the original orientation of the strata at the time of the burrows’ formation. In this case, Skolithos burrows incise cross-beds of various angles, challenging Hydroplate Theory predictions that the cross-beds were tipped during the Compression Event from their original horizontal posture. Photography by James St. John in the public domain under the CC BY 2.0 license. https://commons.wikimedia.org/wiki/File:Skolithos_trace_fossils_in_quartzose_sandstone_ (Mazomanie_Formation,_Upper_Cambrian;_riverside_cliff,_western_side_of_the_St._Croix_River,_northeast_of_ Lookout_Point,_Minnesota,_USA)_5_(18377904824).jpg.
Ichnofossils Associated with Coal Seams
One long-standing question to HPT is the origin of coal seams in a liquefaction submodel. Since earlier editions, Brown (2019) has maintained that coal is the accumulation of plant matter along lenses. As water percolated through sediments after wave-induced liquefaction, vegetation would agglomerate along the lens, further impeding the water’s ascension. As such, these vegetation mats would have formed entirely in subsurface lenses. As Brown (2019, 199) explains:
Each vegetation mat acted as a check valve; that is, during the portion of the wave cycle when water flowed upward, the mat reduced the flow upward through the lens’ roof, so the lens’ volume grew. During the other half of the wave cycle, when water flowed downward, the mat was pushed away from the roof allowing new water to enter the lens. Therefore, water lenses with vegetation mats thickened and expanded during the flood. Vegetation mats became today’s coal seams . . . .
However, as noted by Juby (2011), dinosaur tracks in coal contradict this narrative. Indeed, dinosaur tracks have not only been found in coal but are found with varying associations to coal. In some Utah coal mines, dinosaur tracks often occur in fossil-rich “carbonaceous” sandstone underlying major coal beds (Parker and Balsley 1989). In contrast, some trackways are directly imprinted into the coal, thereby deforming the underlying sandstone (Parker and Balsley 1989; Parker and Rowley 1989). Others still can be found compressing shales into the coal mere centimeters beneath (Peterson 1924). In each case, the tracks are intimately related to the depositional environment of the coal, yet a liquefaction model places these tracks in zones of heavy liquefaction both below and above the parent vegetation mat. In 2011, Brown (2011b, 386) stated he had developed a liquefaction-based explanation for tracks in coal yet to date has not publicly published this explanation, neither has an answer been advanced for tracks in strata both underlying and directly overlying coal. The very concept of coal formation in the liquefaction concept is antithetical to preservation of tracks.
When Could Terrestrial Vertebrate Tracks Be Formed?
The destructiveness of liquefaction has forced Brown to propose that some ichnofossils formed in liquefaction lenses, as discussed above, but such subsurface liquefaction lenses cannot explain the occurrence of tracks from terrestrial vertebrates. This leads to a dilemma: if terrestrial vertebrate ichnofossils could not form in lenses, when could vertebrate traces be produced?
Because ichnofossils would only be preserved if formed in liquefaction lenses, vertebrate traces would have the potential to be preserved if formed after the massive liquefaction during the Compression Event. However, Brown correlates the Compression Event to the wind that encompassed earth’s surface in Genesis 8:1 (Brown 2019, 490, Table 26), the pivotal event that marks the commencement of the recessive stage of the Genesis Flood after the 150th day. By this time, all animals “who had the breath of life in them” had already perished. This relegates the production of terrestrial vertebrate tracks during the Flood to the stages preceding the Compression Event.
Therefore, no additional tracks could be produced by terrestrial vertebrates, regardless of the means, after massive liquefaction during the Compression Event had sorted fauna and destroyed previous ichnofossils. Such tracks could only be preserved if produced in liquefaction lenses, but all terrestrial vertebrates would have already died per Genesis. If the vertebrate track record cannot be formed during this time, then how can the vertebrate bone record be explained by massive liquefaction? This challenges the notion that massive liquefaction produced the succession of terrestrial vertebrate forms and the close correlation between the body fossil record and the track record. Not only would massive liquefaction destroy the vertebrate traces, but it could not explain the succession of vertebrate fossils in the stratigraphic record because that succession was already established, as shown by the close association between the vertebrate track and body fossil records (Lockley 1998; McDonald et al. 2007). Indeed, because tracks had to be formed before the Compression Event, massive liquefaction would have no real bearing on the succession of terrestrial vertebrate fossils and resulting tracks.
Conclusions
As a partial record of geohistory, the stratigraphic record has formed a pivotal centerpiece in the debate on Flood tectonic models. Developed to better understand the initiation and ensuing tectonics of the Genesis Flood, Hydroplate Theory (HPT) has been heralded by some for its simple causal explanation for the stratigraphic record within the biblical paradigm. Although perhaps tangential to the HPT overarching paradigm, the succession of faunal forms and the production of strata based on HPT’s liquefaction postulates continue to be forwarded as one of the strongest cases for HPT, yet this paper has found that case to be internally inconsistent and conflicting with the ichnological record.
Because ichnofossils are merely surficial impressions along or subsurface voids within the substrate, ichnofossils are particularly susceptible to destruction through the reorganization of the sedimentary fabric. As such, HPT struggles to explain ichnofossil production at the surface, coercing HPT to suggest trace fossils were produced in subsurface “liquefaction lenses.” This, however, not only leads to various conceptual contradictions but fails to explain the terrestrial vertebrate ichnological record. Furthermore, the entire terrestrial vertebrate track record and its close correlation to the body fossil record must be produced before the Compression Event and subsequent massive liquefaction, eliminating the need for massive liquefaction to produce faunal succession. The association of tracks with coal seams and geopetal devices cutting across cross-beds are also difficult to explain in HPT’s liquefaction lensing model. Such ichnofossil data challenge HPT’s credibility—a theory purporting to explain the global stratigraphic record and its paleontological constituents. Continued development of HPT is critical to defend its tenets—one that conforms to the empirical record and not conceptual models. If this cannot be done, HPT must be discarded as a possible biblical tectonic model of the Genesis Flood.
References
Ager, Derek V. 1987. The Nature of the Stratigraphical Record. London, United Kingdom: Macmillan.
Ager, Derek. 1993. The New Catastrophism: The Importance of the Rare Event in Geological History. New York, New York: Cambridge University Press.
Alfero, P., J. Delgado, A. Estévez, J.M. Molina, M. Moretti, and J.M. Soria. 2002. “Liquefaction and Fluidization Structures in Messinian Storm Deposits (Bajo Segura Basin, Betic Cordillera, Southern Spain).” International Journal of Earth Sciences 91, no. 3 (May): 505–513.
Austin, Steven A., John R. Baumgardner, D. Russell Humphreys, Andrew A. Snelling, Larry Vardiman, and Kurt P. Wise. 1994. “Catastrophic Plate Tectonics: A Global Flood Model of Earth History.” In Proceedings of the Third International Conference on Creationism, vol. 3, edited by Robert E. Walsh, 609–621. Pittsburgh, Pennsylvania: Creation Science Fellowship.
Boggs, Sam, Jr. 2009. Petrology of Sedimentary Rocks. New York, New York: Cambridge University Press.
Bromley, Richard G., Luis A. Buatois, Gabriela Mángano, Jorge F. Genise, and Ricardo N. Melchor. 2007. “Ichnology: Present Trends and Some Future Directions.” In Sediment—Organism Interactions: A Multifaceted Ichnology, edited by Richard G. Bromley, Luis A. Buatois, Gabriela Mángano, Jorge F. Genise, and Ricardo N. Melchor. Special Publication no. 88. Society for Sedimentary Geology: Tulsa, Oklahoma.
Brown, W. 2011a. “Hydroplate Theory Round 1: Ian Juby (Panelist 1)” In The Flood Science Review, edited by J. Bardwell, 339. Calabasas, California: In Jesus Name Productions.
Brown, W. 2011b. “Hydroplate Theory Round 2: Ian Juby (Panelist 1)” In The Flood Science Review, edited by J. Bardwell, 380–386. Calabasas, California: In Jesus Name Productions.
Brown, W. 2011c. “Hydroplate Theory Round 3: Ian Juby (Panelist 1)” In The Flood Science Review, edited by J. Bardwell, 428–435. Calabasas, California: In Jesus Name Productions.
Brown, Walt. 2019. In the Beginning: Compelling Evidence for Creation and the Flood. 9th edition. Lebanon, Pennsylvania: Mission Imperative Inc.
Buatois, Luis A., and M. Gabriela Mángano. 2011. Ichnology: Organism-Substrate Interactions in Space and Time. Cambridge, Massachusetts: Cambridge University Press.
Clarey, Timothy. 2020. Carved in Stone: Geological Evidence of the Worldwide Flood. Dallas, Texas: Institute for Creation Research.
Cowart, Jack H. and Carl R. Froede Jr. 1994. “The Use of Trace Fossils in Refining Depositional Environments and Their Application to the Creationist Model.” Creation Research Society Quarterly 31, no. 2 (September): 117–124.
Friedman, Gerald M., John E. Sanders, and David C. Kopaska-Merkel. 1992. Principles of Sedimentary Deposits: Stratigraphy and Sedimentology. New York, New York: Macmillan Publishing Company.
Froede, Carl R. Jr., A. Jerry Akridge, and John K. Reed. 2014. “Phanerozoic Animal Tracks: A Challenge for Catastrophic Plate Tectonics.” Creation Research Society Quarterly 51, no. 2 (Fall): 96–103.
Froede, Carl R. Jr. 2010. “Fossilized Animal Tracks and Trackways Date Uplift of the Appalachian Mountains.” Creation Matters 15, no. 4 (July/August): 1, 6–7.
Hildebrandt, C. and S. Egenhoff. 2007. “Shallow-Marine Massive Sandstone Sheets as Indicators of Palaeoseismic Liquefaction—An Example from the Ordovician Shelf of Central Bolivia.” Sedimentary Geology 202, no. 4 (15 December): 581–595.
Holzer, Thomas L. ed. 1998. “The Loma Prieta, California, Earthquake of October 17, 1989—Liquefaction.” U.S. Geological Survey Professional Paper 1551-B: 1–314.
Isaacs, Edward. 2018. “Hydroplate Theory—Problems for Trench Formation in the Pacific Basin.” Journal of Creation 32, no. 3 (December): 58–63.
Isaacs, Edward. 2019. “Edward Isaacs Responds: Hydroplate Theory—Problems for Trench Formation in the Pacific Basin.” Journal of Creation 33, no. 2 (August): 63–64.
Isaacs, Edward A. 2022. “Pre-Tejas Volcanism in North America: Challenge to Hydroplate Theory.” Journal of Creation 36, no. 1 (April): 61–66.
Juby, I. 2011. “Hydroplate Theory Round 2: Ian Juby (Panelist 1)” In The Flood Science Review, edited by J. Bardwell, 375–380. Calabasas, California: In Jesus Name Productions.
Lockley, Martin G. 1998. “The Vertebrate Track Record.” Nature 396, no. 6710 (3 December): 429–432.
Lockley, Martin, Karen Chin, Karen Houck, Masaki Matsukawa, and Reiji Kukihara. 2009. “New Interpretations of Ignotornis, the First-Reported Mesozoic Avian Footprints: Implications for the Paleoecology and Behavior of an Enigmatic Cretaceous Bird.” Cretaceous Research 30, no. 4 (August): 1041–1061.
MacNaughton, Robert B. 2007. “The Application of Trace Fossils to Biostratigraphy.” In Trace Fossils: Concepts, Problems, Prospects, edited by W. Miller III, 135–148. Amsterdam, The Netherlands: Elsevier.
Mángano, M. Gabriela, and Luis A. Buatois. 2017. “The Cambrian Revolutions: Trace-Fossil Record, Timing, Links and Geobiological Impact.” Earth-Science Reviews 173 (October): 96–108.
Mángano, M. Gabriela, Luis A. Buatois, and Robert B. MacNaughton. 2012. “Ichnostratigraphy.” In Trace Fossils as Indicators of Sedimentary Environments, edited by Dirk Knaust and Richard G. Bromley, 195–212. Amsterdam, The Netherlands: Elsevier.
Martin, Anthony J. 2009. “Applications of Trace Fossils to Interpreting Paleoenvironments and Sequence Stratigraphy.” In Fall Line Geology of East Georgia: With a Special Emphasis on the Upper Eocene, edited by M. S. Duncan and R. L. Kath, 35–42, Georgia Geological Society Guidebooks vol. 29, no. 1. Carrollton, Georgia: The Georgia Geological Society.
McDonald, H. Gregory, Richard S. White, Martin G. Lockley, and George E. Mustoe. 2007. “An Indexed Bibliography of Cenozoic Vertebrate Tracks.” New Mexico Museum of Natural History and Science Bulletin 42: 275–302.
Miall, Andrew D. 2016. Stratigraphy: A Modern Synthesis. Cham, Switzerland: Springer International Publishing.
Mitchell, J. D. 2013. Discovering the Animals of Ancient Oregon, 75–91. Southworth, Washington: Leafcutter Press Publisher.
Nickel, Bryan, 2015. “Hydroplate Theory Overview. Part 3.” https://www.youtube.com/watch?v=lThoaW3EVaE.
Nichols, Gary. 2009. Sedimentology and Stratigraphy. West Sussex, United Kingdom: Wiley-Blackwell.
Oard, Michael J. and John K. Reed. 2017. How Noah’s Flood Shaped Our Earth. Powder Springs, Georgia: Creation Book Publishers.
Obermeier, Stephen F., Eric C. Pond, Scott M. Olson, and Russell A. Green. 2002. “Paleoliquefaction Studies in Continental Settings.” In Ancient Seismites: Special Paper, vol. 359, edited by Frank R. Ettensohn, Nicholas Rast, and Carlton Elliot Brett, 13–27. Boulder, Colorado: Geological Society of America.
Parker, Lee R., and John K. Balsley. 1989. “Coal Mines as Localities for Studying Dinosaur Trace Fossils.” In Dinosaur Tracks and Traces, edited by David D. Gillette and Martin G. Lockley. Cambridge, Massachusetts: Cambridge University Press.
Parker, Lee R., and Robert L. Rowley Jr. 1989. “Dinosaur Footprints from a Coal Mine in East-Central Utah.” In Dinosaur Tracks and Traces, edited by David D. Gillette and Martin G. Lockley. Cambridge, Massachusetts: Cambridge University Press.
Pemberton, S. G., J. A. MacEachem, and R. W. Frey. 1992. “Trace Fossil Facies Models: Environmental and Allostratigraphic Significance.” In Facies Models: Response to Sea Level Change, edited by Roger G. Walker and Noel P. James, 47–72. Stittsville, Ontario: Geological Association of Canada.
Peterson, William. 1924. “Dinosaur Tracks in the Roofs of Coal Mines.” Natural History 24, no. 3 (May–June): 388–391.
Reed, John K. 2005. “Strategic Stratigraphy: Reclaiming the Rock Record!” Journal of Creation 19, no. 2 (August): 119–127.
Reed, J. K. 2010. “Untangling Uniformitarianism, Level 1: A Quest for Clarity.” Answers Research Journal 3 (March 17): 37–59. https://answersresearchjournal.org/untangling-uniformitarianism/.
Reed, John K. and Emmett L. Williams. 2012. “Battlegrounds of Natural History: Actualism.” Creation Research Society Quarterly 49, no. 2 (Fall): 135–152.
Sarfati, Jonathan. 2010. “Flood Models and Biblical Realism.” Journal of Creation 24, no. 3 (December): 46–53.
Sassa, Kyoji. 1996. “Prediction of Earthquake Induced Landslides.” Proceedings of the 7th International Symposium on Landslides, vol. 1, 16–21. Trondheim, Norway. Rotterdam, Netherlands: A. A. Balkema.
Seilacher, Adolf. 1967. “Bathymetry of Trace Fossils.” Marine Geology 5, nos. 5–6 (October): 413–428.
Shu, Wenchao, Jinnan Tong, Li Tian, Michael J. Benton, Daoliang Chu, Jianzin Yu, and Wenwei Guo. 2018. “Limuloid Trackways from Permian-Triassic Continental Successions of North China.” Palaeogeography, Palaeoclimatology, Palaeoecology 508 (1 November): 71–90.
Snelling, Andrew A. 2009. Earth’s Catastrophic Past: Geology, Creation & the Flood, vols. 1 and 2. Dallas, Texas: Institute for Creation Research.
Whitmore, John H. 2005. “Origin and Significance of Sand-Filled Cracks and Other Features near the Base of the Coconino Sandstone, Grand Canyon, Arizona, USA.” Creation Research Society Quarterly 42 (December): 163–180.
Whitmore, John H. and Ray Strom. 2010. “Sand Injectites at the Base of the Coconino Sandstone, Grand Canyon, Arizona (USA).” Sedimentary Geology 230, nos. 1–2 (October): 46–59.
Woodmorappe, John. 2006. “Are Soft-Sediment Trace Fossils (Ichnofossils) a Time Problem for the Flood?” Journal of Creation 20, no. 2: 113–122.
Zonneveld, John-Paul, and S. George Pemberton. 2003. “Ichnotaxonomy and Behavioral Implications of Lingulide-Derived Trace Fossils from the Lower and Middle Triassic of Western Canada.” Ichnos 10, no. 1: 25–39.