The views expressed in this paper are those of the writer(s) and are not necessarily those of the ARJ Editor or Answers in Genesis.
Abstract
Precipitation from a warm winter, orographic storm during Christmas of 1996 in Yosemite National Park and the Sierra Nevada was simulated with the NCAR mesoscale weather model called WRF. The sea-surface temperature of the Pacific Ocean upwind of the mountains was prescribed to six different fixed temperatures and the resulting simulated precipitation compared to the actual storm precipitation. Warm sea-surface temperatures increased the precipitation above normal by as much as a factor of four. Based on the likely increase in precipitation rate and frequency of storms following the Genesis Flood, glaciers thousands of feet thick would have developed in a few hundred years.
Keywords: precipitation, Yosemite, Sierra Nevada, winter orographic, weather research and forecasting model, WRF, NCAR, sea-surface temperature, SST, glaciers, Genesis Flood, Pineapple Express
Introduction
Vardiman (2008) proposed that a series of numerical simulations of precipitation be conducted in Yosemite National Park to determine if a warm Pacific Ocean heated by the Genesis Flood could explain the occurrence of glaciers for the Sierra Nevada in a young-earth time frame. He suggested that a conventional mesoscale meteorology model available from the National Center for Atmospheric Research (NCAR) be used to simulate precipitation for several types of storms at multiple sea-surface temperatures (SSTs). The first simulation to be completed was a warm storm with a long, steady fetch over the Pacific Ocean from near Hawaii called The Pineapple Express. The specific storm reported here occurred from December 26, 1996, to January 3, 1997.
General Storm Summary
The Pineapple Express storm is one class of storms which affects the West Coast of the U.S. It consists of a series of storms which travel along a persistent atmospheric flow trajectory from the southwest. It has its origin in the tropical Pacific, frequently near the Hawaiian Islands and lasts from three days to as long as three weeks. Some observers swear they can smell pineapples on the wind during these events. Although the smell of pineapples may be a bit of an exaggeration, the warm, balmy air during a Pineapple Express event is certainly pleasant and moisture laden during normally cold winter storms. When the warm air is lifted over the Coastal Range and the Sierra Nevada along the West Coast, it precipitates massive quantities of rain at low elevations and snow at the high elevations. Often rain falling on snow that has fallen earlier in the winter can cause disastrous flooding along the rivers and lowlands of California, Oregon, and Washington.
It is not uncommon for the total amount of precipitation falling from a series of fronts embedded in such storms to exceed the normal precipitation for an entire season. The author was personally familiar with such a storm that occurred during his tenure as field director of the Sierra Cooperative Pilot Project, a U.S. Department of Interior cloud seeding research project, which dropped almost 30 feet of fresh snow in the central Sierra Nevada near Lake Tahoe in less than a month. The 20-foot tall towers on which rain gages had been installed to measure snowfall in the highest terrain were overtopped by the snow from this single, month-long event.
The heavy precipitation event of December 26, 1996, to January 3, 1997 simulated in this case study was a similar storm. A cool pre-Christmas storm brought valley rain and several feet of mountain snow to southwest Oregon, northern California, and western Nevada on December 21 and 22, 1996. This system set the stage for what would become one of the most historical flood events to affect the region in recent times. A summary of this storm event from which much of the descriptive information for this report is drawn was published online by the NOAA California/Nevada River Forecast Center (Kozlowski and Ekern 1997).
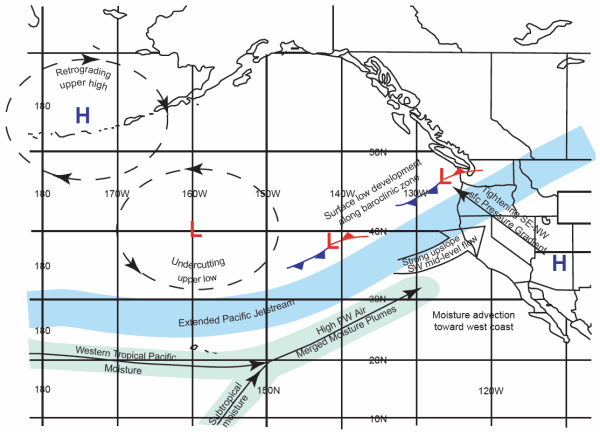
Fig. 1. Flow trajectory and storm tracks for the Pineapple Express case.
Fig. 1 shows the general trajectory of the air flow and storm track over the eastern Pacific and the West Coast of the U.S. for the period of the storm. An upper-level high pressure area over the Aleutian Peninsula and an upper-level low pressure area in the lower Gulf of Alaska forced the polar jet stream southward to flow parallel to the subtropical jet stream. This pattern of strong flow from near Hawaii northeastward across the West Coast of the U.S. persisted for most of the period of the storm.
Sea-Surface Temperature
Fig. 2 shows the distribution of sea-surface temperature over the eastern Pacific Ocean during the Pineapple Express case. The sea-surface temperature of the yellow region over which much of the air flowed before reaching the West Coast for the actual case averaged about 20°C. The source region for the air near Hawaii approached 30°C. The sea-surface temperature was colder close to the coastline because of the clockwise ocean circulation in the Pacific gyre which brings southward some of the colder 10°C water in Gulf of Alaska. The distribution of sea-surface temperature for the simulations was more homogeneous than the actual storm case shown here.
Precipitation
Beginning on Christmas Eve, the overall weather pattern over the Western U.S. began to shift from a polar air mass toward a warmer and wetter tropical regime with promise of a noteworthy precipitation event. Not only would the relentless precipitation through early January 1997 bring widespread flooding, but the snow pack from the pre-Christmas storm would significantly melt to exacerbate problems from the otherwise large amounts of runoff. In the series of tropical air mass storms for this case, precipitation fell across much of the West Coast. The Oregon Climate Services (Gibson, Taylor, and Weisberg 1997) created a graphical re-analysis of the precipitation for the period of December 29, 1996 through January 3, 1997 as shown in Fig. 3. This storm was unique because of the widespread nature of the precipitation footprint.
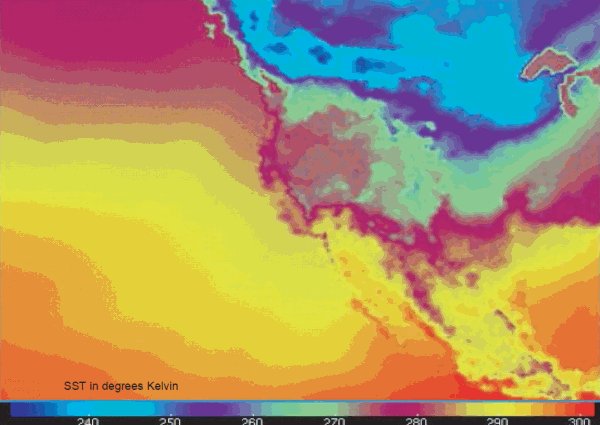
Fig. 2. Distribution of sea-surface temperature in the eastern Pacific for the Pineapple Express case. SST in degrees Kelvin
Excessive precipitation fell over the higher terrain from western Washington southward to northern California and western Nevada. Although the heaviest precipitation for this event was northward of Yosemite National Park, considerable precipitation fell there as well and the air flow over the Sierra Nevada which produced the precipitation was strong even into Southern California. In the simulation study to be described later, the flow over Yosemite was more than sufficient to process and precipitate large quantities of water vapor evaporated from warm sea-surface temperatures.
As the precipitation began to dissipate on January 3, 1997, the 9-day period showed amounts surpassing 40 inches in the Feather River Basin in northern California. Elsewhere across the higher terrain of northern California, amounts ranged from 15 to 30 inches. Between 14 and 18 inches of precipitation fell at the highest elevations along the Sierra Nevada near Yosemite National Park. Table 1 shows selected precipitation totals in California for the 6-day and 9-day periods ending January 3, 1997.
Numerical Simulation of the Pineapple Express
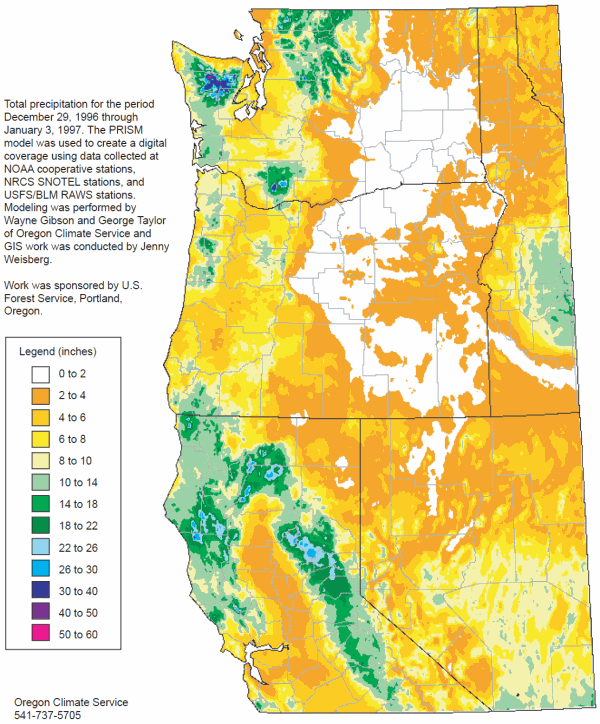
Fig. 3. Total precipitation for the Western U.S. from December 29, 1996 through January 3, 1997 (Gibson, Taylor, and Weisberg 1997).
Vardiman originally proposed simulating storms in Yosemite using the NCAR Mesoscale Meteorology Model (MM5) (NCAR 2003). However, by the time the project began in the Fall of 2008 a new model called the Weather Research and Forecasting Model (WRF) (NCAR 2007) was available with updated capabilities. It is based on similar procedures as MM5 and contains many of the same subroutines. It was decided to use the WRF model for the project rather than the older MM5 model because it has more capabilities and is actively supported.
WRF was installed on the EPIPHANY 44-node parallel processor at the Institute for Creation Research offices in Dallas, Texas, in the fall of 2008. Wes Brewer developed the support software for inputting and storing the data needed for conducting simulations at Yosemite. Topographic data for the Western U.S. and meteorological data for the Pineapple Express case were imported into the model. These data were available from NCAR by exercising subroutines within WRF.
Fig. 4 shows three spatial domains which were established for simulations of the Yosemite cases, all centered on Mt. Hoffmann, a 10,850 foot mountain near the center of Yosemite National Park. The three domains allow simulations to be conducted over progressively larger areas with coarser resolutions. The smallest domain has dimensions about 650 km east/west and 500 km north/south and 3 km grid spacing. The middle domain is three times as large as the inner domain with dimensions about 2,000 km east/west and 1,500 km north/south and a grid spacing of 9 km. The largest domain is nine times as large as the innermost domain with dimensions about 5,600 km east/west and 4,400 km north/south and grid spacing of 27 km. Total storm precipitation in millimeters is displayed by color. The precipitation shown for a sample case in Fig. 4 varied from 0 to over 720 mm (~28 inches).
Validation of WRF for Pineapple Express
WRF has numerous subroutines and parameters which can be activated and adjusted for various conditions. For example, some of the cloud physics subroutines are appropriate for cold, winter storms and others for warm tropical storms. Turbulence and mixing can be adjusted in the dynamics portion of the model. Various radiation codes are available and boundary conditions can be modified. Normally, these subroutines and parameters are adjusted until the computed precipitation from WRF matches the observed precipitation.
The validation method used in the Pineapple Express case study was to set the various parameters in the WRF model according to the conditions expected in winter, orographic storms and compare the computed total storm precipitation with the observed total storm precipitation. Fig. 5 shows a comparison between the computed and actual storm total precipitation for the Pineapple Express case. Table 2 provides additional information for the stations shown on the graph.
The stations in the lower left corner of the graph are generally in the Sacramento Valley at lower elevations and upwind of the Sierra Nevada. The stations in the upper right hand corner are typically at higher elevations and in the mountains, although there are several exceptions. The observed and computed precipitation amounts both tend to increase with altitude, but the relationship is not exact. The regression coefficient between computed and observed total storm precipitation was 0.822. This means that approximately 68% of the observed precipitation over the Sierra Nevada was explained by the WRF model.
This is a good correlation for precipitation measurements, but not excellent. Most efforts to predict precipitation show similar correlations. Fluctuations in cloud coverage throughout a storm and variations in precipitation efficiency often introduce noise in such statistical comparisons. And topographic variation as a function of wind speed and direction relative to the gages, flow patterns, and turbulence also cause large differences in computed and observed precipitation in a gage network with small numbers of instruments. This correlation was judged to be adequate for the purposes of this study.
Table 1. Selected precipitation totals for 6-day and 9-day periods of the December 26, 1996 through January 3, 1997 storm in California.
Northern California 6-Day and 9-Day Precipitation Totals Ending January 3, 1997 | |||
---|---|---|---|
Location | River Basin | 6-Day Totals 12/29/96–01/03/97 | 9-Day Totals 12/26/96–01/03/97 |
Gasquet | Smith | 15.04 inches | 19.84 inches |
Fort Seward | Eel | 8.88 | N/A |
Atlas Peak | Napa | 14.68 | 18.44 |
Venado | Russian | 21.36 | 26.96 |
Lakeshore | Sacramento (Shasta Inflow) | 16.93 | N/A |
Shasta Dam | Sacramento (Shasta Inflow) | 20.04 | 23.70 |
Bucks Lake | Feather | 33.28 | 42.16 |
La Porte | Feather | 30.03 | 40.04 |
Blue Canyon | American | 27.87 | 36.34 |
Auburn Dam Ridge | American | 7.25 | 9.57 |
Sacramento | American | 2.46 | 3.71 |
Gianelli | Stanislaus | 16.46 | 18.66 |
Chilkoot Meadow | San Joaquin | 16.58 | 19.29 |
Fresno | San Joaquin | 2.13 | 2.40 |
Bakersfield | Kern | 0.69 | 1.10 |
Numerical Simulation of Precipitation for Various Sea-Surface Temperatures
The Pineapple Express storm was simulated with the WRF model for six different sea-surface temperatures for the Eastern Pacific Ocean (0°C, 10°C, 20°C, 30°C, 40°C, and 45°C). The purpose was to determine the effect of sea-surface temperature on total storm precipitation in Yosemite National Park. An attempt was made to complete a simulation at 50°C as well, but WRF became unstable and would not complete a run at that temperature even with very small time increments and long runs. The maximum sea-surface temperature possible was at 45°C, for consistent results with moderate time steps and run times. One of the reviewers offered the comment that when running models such as WRF, the parameters are usually adjusted to work well within the range of normally observed temperatures. When sea-surface temperature rises to 30°C or above, the parameters of the model may not be adjusted to such hot temperatures and therefore, one can get spurious results. But, this probably did not happen, as the results seem logical and as expected with such hot sea-surface temperatures. I agree with these comments.
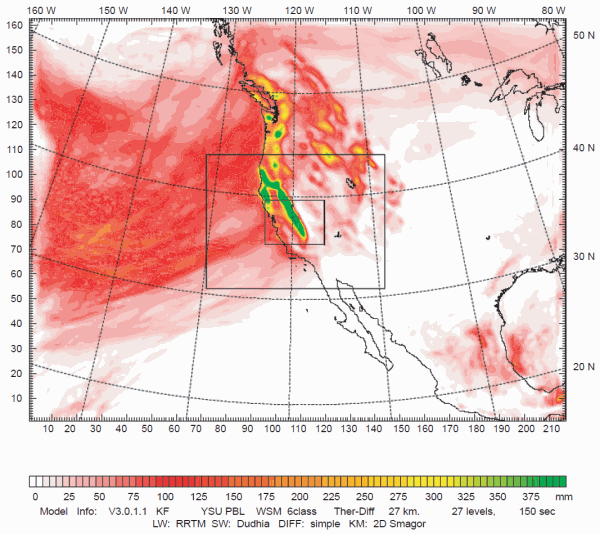
Fig. 4. Three spatial domains established for the simulation of storms in Yosemite National Park. The colored regions represent total storm precipitation in millimeters. This display is the actual storm accumulation for eight days of the Pineapple Express case.
The total 8-day precipitation for the smallest domain is shown in Figs. 6–11 for sea-surface temperatures from 0°C to 45°C. The Pacific Ocean upwind of the California coast was maintained at a constant sea-surface temperature throughout each of the simulations. Note, in Figs. 6–11, the California coastline is shown in the lower left-hand corner of the diagram, the 120° west longitude line (the northeastern border of California) is shown as a dashed, vertical line near the middle, Lake Tahoe is near the top center, and the boundary of Yosemite National Park is shown to the right of the vertical dashed line near the center of the plot.
The size scales of the diagrams are shown in kilometers (km) along the horizontal and vertical axes. The coastal range parallels the California coast about 15 miles inland and varies in elevation from a few hundred feet to a few thousand feet in Southern California. The Sierra Nevada also parallels the California coast, but is located about 150 miles inland and varies in elevation from less than 4,000 feet north of Los Angeles to over 14,000 feet at Mt. Whitney south and east of Yosemite National Park. The ridge line along the Sierra Nevada near Yosemite National Park is generally between 10–12,000 feet.
East of the Sierra Nevada the elevation drops to about 5,000 feet between north-south ridges over the deserts of eastern California, Nevada, Utah, and Arizona. The total precipitation is shown in various shades of color with the color code displayed in millimeters (mm) beneath each figure. The color codes are different for each diagram in order to display the greatest detail.
For the colder temperatures the precipitation patterns show a close relationship between precipitation and the location of mountains. The heaviest precipitation occurred in the Sierra Nevada. At warmer sea-surface temperatures the precipitation seemed to be more controlled by the location of the coast line. The precipitation was more widely distributed over the entire region and less dependent upon elevation.
The precipitation varied from a low of 30 mm (~1 inch) for a sea-surface temperature = 0°C in the San Joaquin Valley upwind of the Sierra Nevada and in the desert to the east to a high of 2,200 mm (~87 inches) for a sea-surface temperature = 45°C along the coast. The maximum precipitation at the warmest sea-surface temperatures displayed along the coast range was not expected. The precipitation over the Sierra Nevada was expected to increase monotonically with warmer sea-surface temperatures. However, precipitation over the Sierra Nevada increased with sea-surface temperature from 0 to about 20°C, then decreased slightly at 30°C before once again increasing at higher sea-surface temperatures.
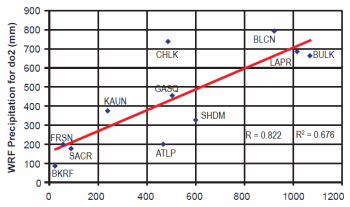
Fig. 5. Comparison of WRF computed precipitation versus Pineapple Express total observed storm precipitation. Data points above the sloping line represent locations where WRF values are larger than observed values and data points below the sloping line represent locations where WRF values are smaller than observed values.
When precipitation in the Sierra Nevada temporarily declined at 30°C, precipitation along the coast range increased dramatically and the valley precipitation upwind of the Sierra Nevada began to increase. It appears that sea-surface temperatures 30°C and warmer over the Pacific Ocean caused convection to break out over the ocean and along the coast. At even warmer sea-surface temperatures precipitation over the ocean and along the coast exceeded precipitation along the Sierra Nevada. Precipitation also became greater over the Sierra Nevada for warmer sea-surface temperatures, but not to the degree of that at the coast.
Table 3 shows a coarse summary of the variation in precipitation as a function of sea-surface temperature and location. The total storm precipitation in the Sierra Nevada increased from about 500 mm by a little over a factor of about 3 to 1700 mm as the temperature increased from 0°C to 45°C with a slight plateau at 30°C. The valley precipitation upwind of the Sierra Nevada increased from 30 mm monotonically by a factor of about 50 over the temperature change from 0°C to 45°C. The coastal precipitation increased from 80 mm monotonically by a factor of about 25 for the same temperature change.
Precipitation Along a Line Perpendicular to the Sierra Nevada
Fig. 12 shows the mid-size model domain with a line 60° relative to north perpendicular to the Sierra Nevada. Fig. 13 shows the precipitation for the Pineapple Express storm from southwest to northeast along the 60° line centered on Mount Hoffman in Yosemite National Park as a function of sea-surface temperature. The 60° line is parallel to the average flow of air over the mountain in the Pineapple Express storm although the flow is more southerly near the surface and more westerly aloft. The 60° line runs from off the coast of Southern California near Monterey, across the Sierra Nevada into Nevada and Utah just south of the Great Salt Lake, ending near Lander, Wyoming. The main peaks in precipitation from left to right are caused by the Coastal Range near the California coast, the Sierra Nevada in eastern California, the Wasatch Range in central Utah, and the Wind River Range in the Rocky Mountains. The set of peaks in precipitation at the left end of Fig. 13 occurs only for the 40°C and 45°C sea-surface temperature and is due to convection over the ocean.
Table 2. Station code, name, elevation, and computed and observed precipitation for the Pineapple Express.
Station Code | Station Name | Elevation (m) | WRF Precipitation (mm) | Observed Precipitation (mm) |
---|---|---|---|---|
BKRF | Bakersfield | 123 | 27.94 | 93.80 |
FRSN | Fresno | 90 | 60.96 | 203.63 |
SACR | Sacramento | 8 | 94.23 | 179.80 |
KAUN | Auburn | 374 | 243.08 | 374.65 |
ATLP | Atlas Peak | 826 | 468.38 | 199.77 |
CHLK | Chilkoot | 2179 | 489.97 | 738.59 |
GASQ | Gasquet | 116 | 503.94 | 455.81 |
SHDM | Shasta Dam | 151 | 601.98 | 328.63 |
BLCN | Blue Canyon | 1429 | 923.04 | 792.84 |
LAPR | La Porte | 1518 | 1017.02 | 686.06 |
BULK | Bucks Lake | 1573 | 1070.86 | 663.18 |
Notice that the precipitation increases with sea-surface temperature over each of the mountain ridges. It also increases more strongly at higher temperatures. This will be discussed more fully in the next section. Between the mountain ridges the precipitation remains relatively low, particularly in the deserts of Nevada and Utah. However, over the Pacific Ocean off the coast of California the precipitation increases strongly with sea-surface temperature although there is no orographic influence. This widespread precipitation was noted earlier in the horizontal displays of precipitation for high values of sea-surface temperature and is believed to be due to instability over the warm ocean producing convection. This instability also extended onto the continent upwind and onto the coastal range and into the Central Valley of California. Notice that at the highest sea-surface temperatures the precipitation increased uniformly all the way from the farthest southwest to Mount Hoffman.
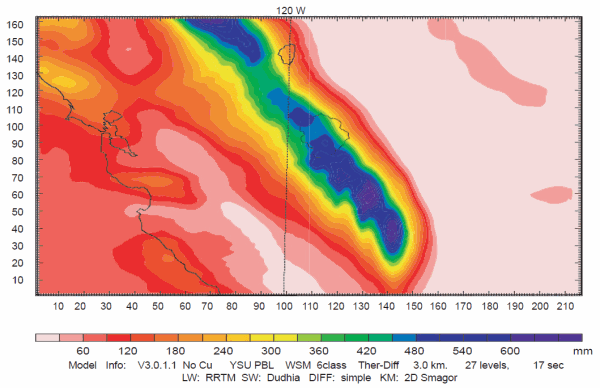
Fig. 6. Total Pineapple Express storm precipitation for sea-surface temperature = 0°C.
Accumulation of Precipitation in Yosemite National Park as a Function of Sea-Surface Temperature
Fig. 14 displays the accumulated WRF model precipitation as a function of simulation time and sea-surface temperature. The accumulated precipitation shown here is the maximum precipitation at all grid points within the Yosemite National Park boundary shown in Figs. 6–11. The precipitation accumulates more rapidly for the warmer sea-surface temperatures indicating a higher precipitation rate at warmer temperatures, as would be expected. The WRF computed precipitation for the actual storm (indicated as default) occurs between the 20°C and 30°C cases. This would be expected since the sea-surface temperature for the actual storm averaged about 22°C, although its sea-surface temperature was not distributed homogeneously over the Eastern Pacific like the other six cases.
Note, that the accumulation curves for the various sea-surface temperatures do not increase regularly as expected. For example, the 30°C accumulation curve falls below the actual case and the 20°C curve between about 125 and 175 hours before climbing rapidly back to the expected order before the end of the storm. Why the model does this is unclear. However, by the end of the storm, the order of all sea-surface temperatures is as one would expect.
The difference in the accumulated precipitation is greater at higher temperatures, for example, the difference is about 20 mm between 0°C and 10°C, 115 mm between 10°C and 20°C, 420 mm between 20°C and 30°C, 500 mm between 30°C and 40°C, and 850 mm between 40°C and 45°C. This would be expected since the rate of growth of snow in clouds and the formation of precipitation should be proportional to the water vapor available for cloud processes. But, the amount of water vapor is an exponential function of sea-surface temperature as governed by the Clausius- Clapeyron equation. This is a well-known chemical-physics equation that relates water vapor pressure in air to the temperature of a nearby water surface.
According to this logic the accumulated precipitation should be an exponential function of sea-surface temperature. Under the same storm conditions, the accumulated precipitation in Yosemite National Park should be about two times greater at 30°C than at 0°C and four times greater at 45°C than at 0°C. Consequently, when the surface of the Eastern Pacific is warm, large amounts of snow will accumulate in the Sierra Nevada and when it is cold only small amounts will accumulate, as shown in Fig. 14.
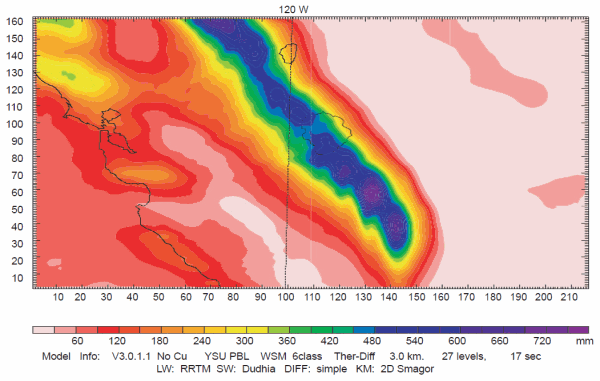
Fig. 7. Total Pineapple Express storm precipitation for sea-surface temperature = 10°C.
Increased Glacier Growth
This study has shown that precipitation can be doubled or even quadrupled in the Sierra Nevada if the sea-surface temperature of the Pacific Ocean is warmer. Such a large increase in precipitation would readily lead to larger, more permanent glaciers if the precipitation fell as snow. However, Pineapple Express type storms occurring under warm sea-surface temperatures could have a higher snow level. That is one of the dangers of such storms today—the likelihood of rain at high elevations falling on snow producing flooding. Would a warm ocean cause the snow level to be even higher than such storms of today? If they did, then larger, thicker glaciers could be eroded quickly under such conditions. It seems likely that more rain will fall on the Coastal Range of California and the foothills of the Sierra Nevada under warmer sea-surface temperatures, but snow will remain the dominate precipitation type at higher elevations. Although a warmer ocean will moderate the climate much as it does today, it would likely extend its temperature influence only a relatively short distance inland. Today, this effect typically extends less than about 50 km inland. Mount Hoffman in Yosemite National Park is about 200 km from the coastline. So, even if the moderating effect of the ocean were doubled to 100 km, the region where most glaciers occur would still be outside the direct warming influence of higher sea-surface temperatures. Of course, Pineapple Express type storms would still tend to produce rain on snow as they do today and melt some of the snow.
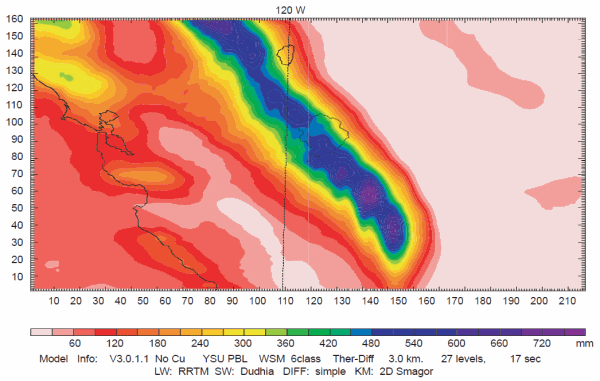
Fig. 8. Total Pineapple Express storm precipitation for sea-surface temperature = 20°C.
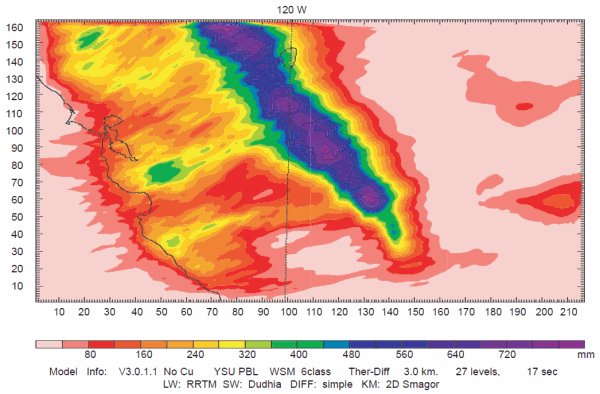
Fig. 9. Total Pineapple Express storm precipitation for sea-surface temperature = 30°C.
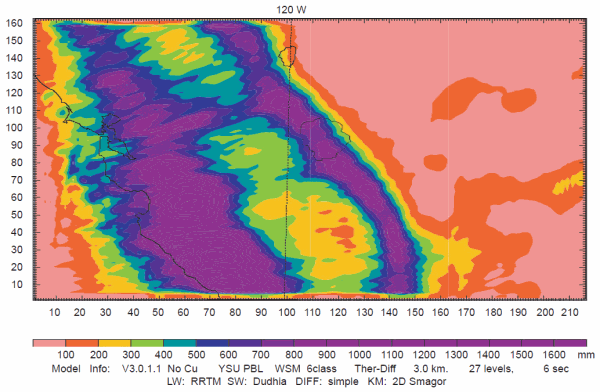
Fig. 10. Total Pineapple Express storm precipitation for sea-surface temperature = 40°C.
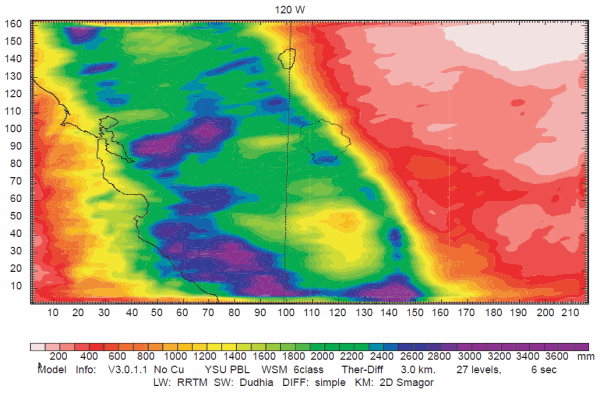
Fig. 11. Total Pineapple Express storm precipitation for sea-surface temperature = 45°C.
One of the reviewers suggested a possible mechanism for keeping the snow from melting in the Sierra Nevada even with warm advection from the Pacific. Volcanic aerosols in the stratosphere would likely have been prevalent following the Genesis Flood and would have kept the interior of the continents cool, even in summer, and the Sierras would have tended to have a cool low-level flow from the east. So, as warm low pressure systems approached from the west, winds from the east and southeast ahead of the storm would have caused low-level air (below 12,000 feet above sea level) to spread into the Sierras. Warm precipitation would start out as heavy snow (riding over the cooler low-level air), change into freezing rain, and then rain when the wind shifted out of the west. But, the rain would simply be absorbed into the snowpack, and as the effect of warm westerly winds waned after a day or so, the water in the snow pack would then freeze. So, volcanic aerosols would likely be needed, especially in summer, to keep the snow from melting at high altitudes.
Table 3. Total storm precipitation as a function of sea-surface temperature and location.
Sea-Surface Temperature | (°C) Coastal Precipitation (mm) | Valley Precipitation (mm) | Sierra Precipitation (mm) |
---|---|---|---|
0 | 80 | 30 | 500 |
10 | 100 | 60 | 600 |
20 | 150 | 100 | 800 |
30 | 400 | 150 | 700 |
40 | 800 | 400 | 900 |
45 | 2200 | 1500 | 1700 |
An effect that was not modeled in this study is the increased frequency of colder storms coming from the northwest during ice age conditions. Crowley and North (1991) have shown that the path of the jet stream was positioned much further south during the ice age—crossing from the Pacific Ocean onto the North American continent near San Francisco. Such a position would have caused the frequency of storms crossing the Sierra Nevada to be increased greatly, probably doubling the number of storms which dumped rain and snow each winter. Furthermore, the storms would probably have been colder due to cold, polar air being displaced farther south. And the winter season would have been extended into the summer reducing the melting of glaciers. Multiplying the two effects together—the increase in precipitation due to warmer sea-surface temperatures and the greater frequency of cold storms—it is likely that the snowfall in the Yosemite National Park and the Sierra Nevada in general, would have been four to eight times what it is today and less of the glaciers would have melted in the summer. A full assessment of the difference in glacial growth must wait until additional types of storm cases have been simulated, but a crude estimate will be attempted here.
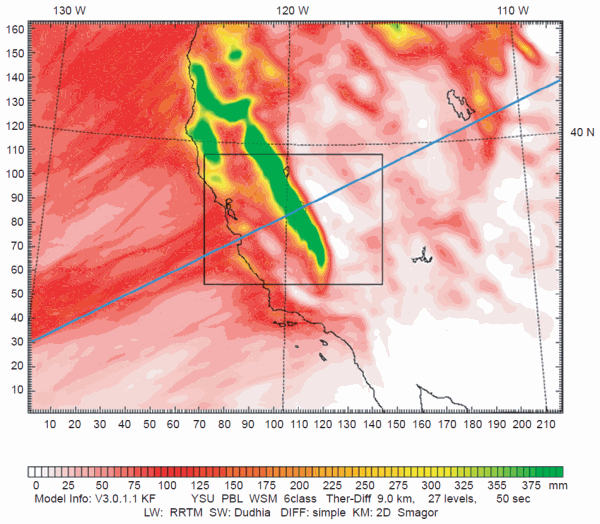
Fig. 12. Mid-size domain with a 60° line perpendicular to the Sierra Nevada. The colors indicate total accumulated precipitation in millimeters. This display is for accumulated precipitation after eight days for the actual Pineapple Express case.
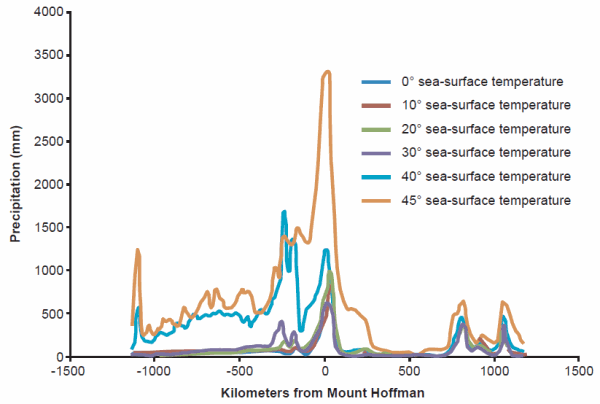
Fig. 13. Precipitation for the Pineapple Express storm from southwest to northeast along the 60° line in Fig. 12 centered on Mount Hoffman as a function of sea-surface temperature.
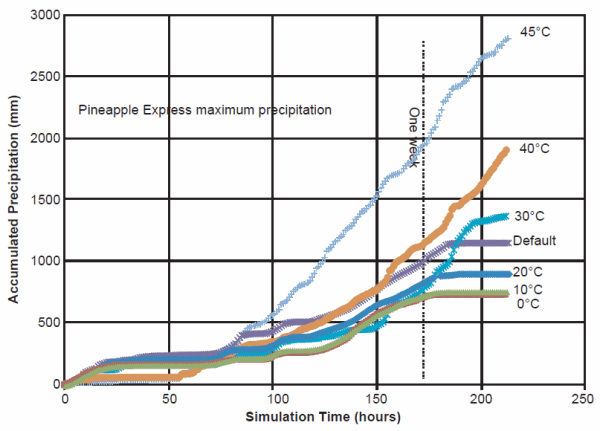
Fig. 14. Accumulated WRF model precipitation in Yosemite National Park as a function of simulation time and sea-surface temperature.
Fig. 14 shows that a Pineapple Express type storm will precipitate a total of between 700 and 2,000 mm per week depending upon sea-surface temperature. Considering precipitation only from this type of storm it is possible to calculate the depth of glaciers that will accumulate in Yosemite National Park if no melting occurs in the summers. For example, one Pineapple Express type storm which precipitates 1,000 mm will contribute about 3.3 feet of ice to a glacier. Snow would be five to ten times greater in depth, but it would be compressed to the density of ice over time. The glacier thickness calculated here will be for ice. If only one Pineapple Express storm occurred each year, the glacier would be 330 feet thick in 100 years. However, if a Pineapple Express type storm occurred every four weeks, the glacier would be over 4,000 feet thick in 100 years.
The WRF model computes precipitation in water equivalent millimeters, i.e., the depth of rain in millimeters or the depth snow would have in millimeters if it fell as rain. But, snow often initially has a density only 10% to 20% that of water. Snow is compressed by the accumulation of additional precipitation falling on the surface above. Its density increases to that of solid ice, eventually to about 90% that of water. Consequently, 1,000 millimeters of water equivalent precipitation in the form of snow that doesn’t melt will eventually contribute slightly more than 1 meter or 3.3 feet to the depth of a permanent glacier.
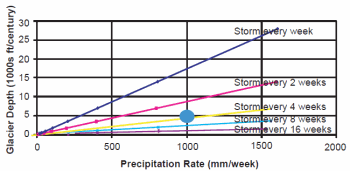
Fig. 15. Glacier depth as a function of precipitation rate and storm frequency.
Fig. 15 shows glacier depth as a function of precipitation rate and frequency of storms in Yosemite National Park. Notice that glacier thickness is a function of precipitation rate, frequency of storms, and the length of an ice age. The blue oval in Fig. 15 represents an average condition which could have occurred during an ice age with a warm ocean. If a storm like the Pineapple Express storm occurred on average once every four weeks with a precipitation rate of 1,000 mm per week, a glacier 5,000 feet thick would have developed in approximately 100 years. Since precipitation rate is a function of sea-surface temperature and storm frequency is a function of the location of the jet stream, it would appear that the presence of glaciers in Yosemite National Park during an ice age can easily be explained by warm sea-surface temperatures and a more southerly position of the jet stream. Some melting of the glaciers would likely occur depending on the length of the summers. Other types of storms will also contribute to the growth of the glaciers. The length of an ice age is also important to the maximum depth of the glaciers. When additional case studies are completed for other storm types, a more detailed glacier model will be developed.
It is likely that Pineapple Express-like storms coming from directions other than the southwest like they do today could have also produced heavy snowfall. For example, a consistent flow from the west is common today, but does not produce large accumulations of snow in the Sierra Nevada because the underlying ocean surface is cold and large quantities of moisture are not evaporated into the atmosphere. If such continuous flow patterns from the west, or even northwest, were to flow over a warm Pacific Ocean, heavy accumulations like Pineapple Express storms could have added significantly to the annual snowpack.
Conclusions
A warm, tropical storm during the Christmas holidays of 1996–1997 called the Pineapple Express storm has been successfully simulated. Additional simulations of warm sea-surface temperatures in the eastern Pacific Ocean were conducted to find if precipitation in Yosemite National Park would have been increased. Warm sea-surface temperatures doubled or quadrupled the precipitation in Yosemite National Park and throughout the Sierra Nevada. This enhanced snowfall and greater frequency of storms appear to be adequate to explain glaciation in the Sierra Nevada during an ice age in a young-earth time frame. Glaciers thousands of feet thick could have readily developed during hundreds of years following the Genesis Flood.
Acknowledgments
The mesoscale meteorology model (WRF) used in this study was developed and maintained by the National Center for Atmospheric Research (NCAR) available at http://www.wrf-model.org/index.php. The meteorological and topographic data (NARR) used in this study were provided by the National Oceanographic and Atmospheric Administration (NOAA) National Operational Model Archive & Distribution System available online at http://www.emc.ncep.noaa.gov/mmb/rreanl/. Richard Carpenter and Brent Shaw of Weather Decision Technologies, Inc., and Valentine Anantharaj and Xingang Fan of Mississippi State University offered occasional assistance in the use of WRF. This research was funded by the National Creation Research Foundation of the Institute for Creation Research.
References
Crowley, T. J. and G. R. North. 1991. Paleoclimatology. Oxford Monographs on Geology and Geophysics no. 18, pp. 71–91. New York: Oxford University Press.
Gibson, W., G. Taylor, and J. Weisberg. 1997. Oregon climate service. Retrieved from, http://www.cnrfc.noaa.gov/images/storm_summaries/jan1997/misc/jan_flood.gif.
Kozlowski, D. and M. Ekern. 1997. Retrieved from, http://www.cnrfc.noaa.gov/storm_summaries/jan1997storms.php.
National Center for Atmospheric Research. 2003. Retrieved from, http://www.mmm.ucar.edu/mm5/.
National Center for Atmospheric Research. 2007. Retrieved from, http://www.wrf-model.org/index.php.
Vardiman, L. 2008. A proposed mesoscale simulation of precipitation in Yosemite National Park with a warm ocean. In Proceedings of the sixth international conference on creationism, ed. A. A. Snelling, pp. 307–319. Pittsburgh, Pennsylvania: Creation Science Fellowship; Dallas, Texas: Institute for Creation Research.