The views expressed in this paper are those of the writer(s) and are not necessarily those of the ARJ Editor or Answers in Genesis.
Abstract
The highly energetic geological processes inevitably accompanying the Genesis Flood must have generated an enormous heat load without raising environmental temperatures beyond biological endurance limits. This is the fourth in a series of papers intended to identify and, where possible, to quantify the key sources of Flood heat. Our primary concern in this paper is with the heat deposited as a result of Flood and post-Flood magmatic activity. Most of this heat must have been removed from earth’s surface rocks and biosphere within at most a few hundred years. Related preliminary questions considered here include global energy totals, the relative importance of plumes and plates in mantle convection, the significance of two enormous mantle heterogeneities on the core-mantle boundary, the onset of plate tectonics, and the thermal history of the mantle. For future reference the relevant literature is briefly reviewed for each of these topics.
The ocean floors represent by far the largest source of magmatic heat released during and since the Flood. Although this cooling problem was addressed by Worraker and Ward (2018), no solution compatible with a biblical timescale was found. Here possible ways of modifying and extending that work are considered, including variations in model input parameters and the proposal that rapid cooling might have been accomplished by 4D thermal radiation. Diffusion chronometry, an important technique for estimating the duration of geochemical processes, is considered in relation to cooling times, which are often found to be shorter than expected within the uniformitarian paradigm. Other field evidence of cooling modes and timescales is also considered. Large Igneous Provinces (LIPs) are next in importance. After describing their general characteristics, the heat deposited in the formation of LIPs is estimated; in terms of the thermal capacity of the oceans this is overwhelming. Although this heat is insignificant in comparison with ocean floor heat as a whole, potentially it could have produced drastic local effects. LIP eruption rates and eruption patterns are then discussed, followed by their emplacement and cooling modes, and the timescales involved. Diffusion chronometry again figures as important in estimating cooling times.
Our main conclusion is that the heat deposited in the formation of the ocean floors and of LIPs is overwhelmingly large and cannot be removed by known natural processes within a biblically compatible timescale. We have noted, however, that this is only a problem for our limited understanding of the processes at work during the Flood, which very probably involved supernatural intervention: God was in full control of every aspect throughout. The literature on the thermal history of earth’s mantle indicates a general cooling of approximately 150°C since the onset of the Flood; the range of mantle potential temperatures at any one stratigraphic level has been ~170°C throughout. However several potentially fruitful lines of further investigation are suggested.
Keywords: mid-ocean ridge; oceanic lithosphere; potential temperature; diffusion chronometry; large igneous provinces
Introduction
The catastrophic global Flood at the time of Noah (described in Genesis 7 and 8) must have generated an enormous quantity of heat, thus raising the question of how environmental temperatures were kept within limits. This article is the fourth in a series aiming to identify, and where possible to quantify, the sources of Flood heat in order to provide boundary conditions and guidelines for creation scientists seeking to explain how the necessary cooling was accomplished.
The first paper (Worraker 2018) reviewed boundary conditions relevant to modelling the earth’s thermal history. The only ocean temperature indicator considered was the oxygen isotope ratio (18O/16O) in fossil shells and in ice, expressed as the δ18O value. Part 2 (Worraker 2019) considered other widely-used temperature indicators, viz. (1) the Mg/Ca (magnesium/calcium) ratio in calcite fossil shells; (2) trace element methods, for example, Sr/Ca and Li/Mg ratios in corals; (3) biomolecular index methods; (4) the carbonate clumped isotope index. Part 3 (Worraker 2020) reviewed vapor canopy models, in which the atmosphere of the pre-Flood earth is characterized by a high-altitude vapor canopy. The present article, Part 4, considers the geological heat deposited in the formation of igneous and metamorphic rocks during and after the Flood, the largest sources being the ocean floors and Large Igneous Provinces (LIPs). Our interest is not only in the total quantity of heat deposited, but also in the processes by which it may have been removed from crustal rocks and the biosphere and in the time taken to complete these processes. Heat deposited in the formation of continental rocks is not considered here, as most of the continental crust was probably formed during the first part of Creation Week prior to the creation of plant life (Genesis 1:11–12) on Day 3; see Baumgardner (2000).
Accelerated Nuclear Decay (AND) was identified in the RATE (Radioisotopes and the Age of the Earth) project as a major source of heat during the Flood (Vardiman et al. 2005; Worraker 2016; 2018), but it is purposely excluded from consideration in the present article in order to focus attention on the heat released in the formation of igneous and metamorphic rocks without nuclear complications.1 Questions related to the AND phenomenon and its impact are to be addressed in Part 5 of this series. The magnitude and effect of the heat load due to impacts from space, including asteroids and comets, are to be considered in Part 6. The final planned article, Part 7, is intended to summarize the conclusions of the whole series, to provide suggestions for follow-up creation science research, and to deal with the important question of supernatural involvement in the events of the Flood and afterwards: to what extent, and in what ways, was God active during the Flood? We touch briefly on this question in our conclusions here, but plan to deal with it more fully in Part 7.
Since there is no unanimity in Flood modelling in present-day creation science, it is useful to choose a reference model framework. For this purpose the Catastrophic Plate Tectonics (CPT) model is the natural choice as the most widely accepted and most highly developed Flood model among creation scientists; indeed it is the only model which has been subject to serious quantitative investigation. However, CPT is not accepted by all, and its use here as providing a reference Flood scenario is not intended to signify its acceptance as the final word in Flood modelling. Although there is considerable remaining controversy over stratigraphic placement of the end of the Flood in the geological record, that issue has no impact on the heat problem addressed here as our interest includes the entire Flood and post-Flood periods. Furthermore, stratigraphic placement of the onset of the Flood is not a major issue here either since the present-day ocean floors, which account for most of this heat, formed relatively late in the Flood. Also, by far the largest known LIPs are found in Cretaceous rocks, implying formation well within the Flood period.
Having outlined the most relevant features of CPT, we give a perspective on global energies, followed by an appraisal of the thermal history of the mantle abstracted as far as possible from the uniformitarian timescale assumed in the conventional literature; this provides an important backdrop for Flood modelling. Since mantle thermal history is inevitably interwoven with other contentious geological issues, notably the relative importance of plumes and plates in mantle convection, the significance of mantle heterogeneities and the timing of the onset of plate tectonics, Appendices 1 and 2 summarize these other topics and their history as presented in some of the conventional literature. Although none of these topics (mantle thermal history; plumes and plates together with mantle heterogeneities; the onset of plate tectonics) is central to the immediate question of magma cooling, they are all interrelated and are all likely to figure in a comprehensive creation science understanding of geological history. The coverage here is intended to provide an introduction to the relevant literature for future reference.
We then consider the main sources of geological heat related to igneous and metamorphic rock formation. This involves quantifying the heat deposition as far as possible and assessing possible ways of accomplishing the necessary cooling together with the available evidence in the rocks for the modes and timescales of cooling. By far the largest heat source is the formation of the ocean floors, which in the long-age framework are generally no older than 200 Ma; their formation has been taking place between the stages in the Flood corresponding to Jurassic deposits (possibly earlier, since earlier deposits have almost certainly been buried by subduction) and the present. This problem was addressed on the ocean basin scale by Worraker and Ward (2018) using simple plate modelling, but these authors could not find a satisfactory solution. Here we consider various other aspects of ocean floor cooling: (1) the impact of parameter variations in the model used by Worraker and Ward (2018); (2) the possibility of thermal radiation in a fourth spatial dimension as the means of rapid cooling; (3) other constraints on the cooling process from available field evidence. Diffusion chronometry, a widely-used method of estimating the duration of various geophysical/geochemical processes, is introduced in Appendix 4. It is considered here as it is applicable to a wide range of minerals and often gives results at odds with methods based on radioactive decay series, yet it has hitherto received little attention from creation scientists except in particular cases such as helium diffusion in zircons (Humphreys 2005). Potentially fruitful lines of further investigation relevant to ocean floor cooling are noted for future reference.
LIPs are considered next. Although these are found practically throughout the geological record, our concern is primarily with those emplaced from the Cambrian onwards. Since LIPs have hitherto attracted little interest from creation scientists except in certain special cases (for example, Oard 1999; Woodmorappe and Oard 2002), these major geological formations and the magmatic activity they represent are described and considered in some detail. The heat deposited in the formation of LIPs is estimated, and the data relating to their emplacement and cooling are considered with a view to assessing cooling mechanisms and timescales. Again, potentially fruitful lines of follow-up investigation are suggested. Our conclusions follow, together with recommendations for further work.
Geological times (that is, dates and time intervals) from the conventional mainstream literature are for convenience cited as reported, either in millions of years (Ma) or billions of years (Ga), but this does not imply acceptance of the reality of these times.
The Catastrophic Plate Tectonics Model and its Implications
In 1859 Antonio Snider-Pellegrini proposed that rapid, horizontal divergence of crustal plates had occurred during Noah’s Flood (Austin et al. 1994). Thus, plate tectonics theory was first elaborated in terms of flood geology; not until about 100 years later was plate tectonics generally accepted within the dominant uniformitarian geological consensus. In a more modern context, serious modelling of CPT as a fundamental mechanism underlying the Genesis Flood seems to have been initiated by Baumgardner (1986). A more general, more formal presentation is given by Austin et al. (1994). Their description of CPT includes the following features of particular relevance here:
- At the beginning of the Flood, the earth is divided internally into core, mantle, and crust, essentially as it is today, though the mantle is postulated to have been less viscous than it is now. The crust is differentiated horizontally between stable, sialic, cratonic crust on the continents, and denser mafic (probably basaltic) ocean crust;
- Although no specific initiating event is proposed, the major action begins with slabs of oceanic crust breaking loose and subducting along thousands of kilometers of pre-Flood continental margins. The subducting slabs deform and thus heat the mantle locally, which reduces the viscosity locally, in turn leading to a faster subduction rate. Thus, a positive feedback loop is established between increasing subduction rate, increasing dissipative heating, and decreasing local viscosity, leading to subduction rates measured in meters per second;
- The above thermal runaway mechanism produces several important consequences, notably (1) global overturning of the mantle; (2) a major rise in sea levels and hence the inundation of the continents; (3) continental collisions; (4) the formation of long ocean-floor spreading centers caused by subduction-induced stretching of the ocean crust. At these spreading centers upwelling mantle material degasses its volatiles and vaporizes ocean water. This would produce linear geysers of superheated gases along the whole length of the spreading centers. These gases would cool by radiation into space, the water they contain condensing and falling as intense global rain;
- Thus the fountains of the great deep and the windows of heaven (Genesis 7:11 and 8:2) are interpreted in this CPT model in terms of the above-postulated linear geysers; water displaced onto the continents by the freshly raised ocean floors is also suggested as a possible water source. Austin et al. (1994) also suggest, referring to Genesis 7:24–8:2, that the latter would have remained a source of water for up to 150 days;
- Rapid plate motion would have ceased when virtually all the pre-Flood oceanic floor had been replaced with new, less-dense, less-subductible rock. The lack of new, hot mantle material would have stopped the spreading-center geysers and resulting global rain. Austin et al. (1994) refer this point in time to Genesis 8:2;
- Warm oceans immediately after the Flood follow naturally from this CPT scenario, and form an essential part of Oard’s (1990) model of an Ice Age in a young-earth framework. Present-day isostatic crustal movements, earthquakes and volcanoes also follow naturally as representing “relict Flood dynamics.”
Some details of these features of CPT as proposed in 1994 have since needed modification in the light of subsequent research. In particular, the runaway mechanism outlined in point 2 above, which depends on viscosity reduction with rising temperature, is now understood to result from strain weakening rather than thermal weakening (Baumgardner 2003; Cho et al. 2018). Also, the global rain which fell during the Flood is no longer thought to result mainly from the condensation of steam from geysers along the mid-ocean ridges, but rather from ocean water carried aloft by the steam jets subsequently falling back to earth (Baumgardner 2003).
Background Geological Issues
The most important background issue here is the thermal history of the mantle, but for the sake of perspective we first consider the magnitudes of global energies. Although radiogenic heat is potentially important in this context, in practice it does not play a major role in the processes of greatest concern, viz. the cooling of the ocean lithosphere and of LIPs. This is because the concentration of heat-producing radionuclides in mafic and ultramafic rocks, which constitute the bulk of these formations, is relatively low as can be seen in Stacey and Davis (2008), table 21.3; see also Baumgardner (2000); Furlong and Chapman (2013). As it is planned to consider radiogenic heating of the earth in some detail in Part 5 of this series, it will not be discussed further here.
Perspectives on the earth’s global energies
Before considering particular categories of igneous rock formation and the associated heat problems, we present an overview of the various components of the earth’s global energies. Most entries in table 1 are based on the figures given in table 21.1 of Stacey and Davis (2008, 349), plus some additional items of particular interest here.
Description | Energy (× 1030 J) |
---|---|
Gravitational binding energy* | –249.0 |
Accretion energy | 219.0 |
Core separation (minus elastic energy) | 13.9 |
Inner core formation | 0.09 |
Mantle differentiation | 0.03 |
Elastic strain energy | 15.8 |
Radiogenic heat generated in 4.5 × 109 years | 7.6 |
Residual stored heat | 13.3 |
Heat lost in 4.5 × 109 years | 13.4 |
Rotational energy | 0.2 |
Tidal dissipation in 4.5 × 109 years# | ~1.1 |
Heat released in ocean lithosphere formation† | 0.14 |
Solar energy incident in 1 year†† | 5.5 × 10–6 |
Note that some of the table entries are described in terms presupposing the conventional uniformitarian picture of the earth forming 4.5 billion years ago by the accretion of planetesimals from a disk of material believed to have surrounded the early sun, followed by early internal segregation and core formation (for example, Chambers 2004; Taylor 2001; Wood, Walter, and Wade 2006). The gravitational binding energy, which is negative, represents the energy required to pull apart all of the earth’s material to infinity against its mutual gravitational attraction. It is numerically equal to the sum of the following five table entries. The very large entry labelled “accretion energy” is the energy that would have been released in the uniformitarian picture of origins as the material forming the earth was drawn together by gravity. This would have been manifested as heat: Stacey and Davis (2008, 349) estimate that if all this energy had been retained, the earth would have possessed an average temperature exceeding 37,000 K! However they argue that most would have been radiated away during the accretion process, and maintain that the accretion energy, not the energy released by radioactivity, is the prime reason why the earth’s interior is hot. The entry for elastic strain energy represents the non-recoverable energy due to gravitational compression of the earth’s constituent materials.
Note that any radial redistribution of material within the earth, which involves work done by or against gravity, will lead to changes in the earth’s gravitational binding energy, and in other energy totals too, viz. the elastic strain energy and rotational energy. Thus processes such as the sinking of dense ocean lithosphere and the surfacing of hot magma and its subsequent cooling and contraction will affect these energy totals and, through the resulting changes in the earth’s moment of inertia, the length of earth days (Stenberg 2012). However, the consensus in the conventional literature is that the earth’s rotation rate has been controlled largely by tidal effects within the earth-moon dynamical system (for example, Coughenour, Archer, and Lacovara 2009; Tyler 2021; Williams 2000); the length of the day was supposedly 21.5 hours about 400 Ma ago (corresponding to Devonian strata), implying considerable change through earth history. By comparison, effects due to changes in the distribution of material within the earth seem to have been inconsequential. Although these conclusions depend at least in part on uniformitarian assumptions, detailed analysis would be a complex exercise beyond the scope of this article and involving many uncertainties. Hence these effects are ignored here.
Thermal history of the mantle
Papers 1 and 2 of this series (Worraker 2018; 2019) considered what is known about the thermal history of the oceans in terms of the data recorded in various temperature indicators or proxies. However, a further aspect of the earth’s thermal history relevant to the deposition of geological heat is the thermal history of the mantle. Radial (that is, 1D) profiles of the present-day globally-averaged temperature and pressure fields within the earth according to the Preliminary Reference Earth Model (PREM) of Dziewonski and Anderson (1981) are shown in fig. 1 in Worraker (2018). Other features of the earth’s internal structure noted by Worraker (2018) include (1) thermal boundary layers just above the Core-Mantle Boundary (CMB) and between the transition zone (at 410 km depth) and the surface, and (2) mantle heterogeneities. Some of these heterogeneities are very large and extend down into the lower mantle, for example the two Large Low Shear-Wave Velocity Provinces (LLSVPs) on the core-mantle boundary, known colloquially as Tuzo (beneath Africa) and Jason (beneath the Pacific Ocean), which were discovered seismically; they are illustrated here in fig. 1. For further details see Appendix 1.
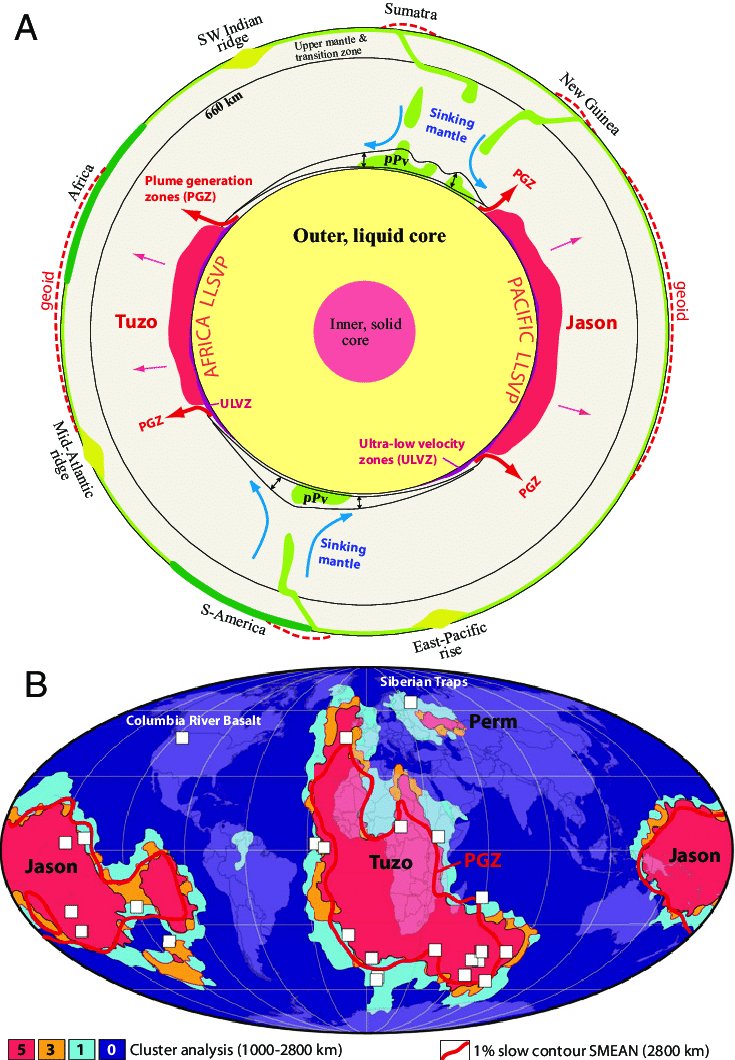
Fig. 1. Reproduction of fig. 1 in Torsvik et al. (2014). (A) Schematic cross section of the earth as seen from the South Pole. The earth’s lower mantle is dominated by the two antipodal Large Low Shear Wave Velocity Provinces (LLSVPs) beneath Africa (Tuzo) and the Pacific (Jason). These dominate the elevated regions of the residual geoid (dashed red lines), and their margins. Plume generation zones (PGZs), are thought to be the principal source regions for Large Igneous Provinces and kimberlites. The thin arrows above Tuzo and Jason indicate that the residual geoid is apparently a result of buoyant upwellings overlying these mantle structures. The “pPv” (between the two lines separated by up-down arrows) indicates lenses of post-perovskite. (B) Reconstructed Large Igneous Provinces for the past 300 Ma and the 1% slow contour (2800 km depth) used as a proxy for the plume generation zones. Also shown are the “voting” map contours of Lekic et al. (2012). Contours 5–1 (only 5, 3, and 1 are shown for clarity) define Tuzo and Jason in addition to a smaller Perm anomaly. The Columbia River Basalt (17 Ma) is the only anomalous Large Igneous Province (located above faster regions, contour 0) in these global tomographic models.
Our particular interest here is in the thermal history of the mantle through the record embodied in igneous and metamorphic rocks. The natural uniformitarian expectation, given the assumed very hot conditions in the early earth and the secular decline in radiogenic heat production, is that the mantle must have cooled progressively through geological time (for example, Abbott et al. 1994; Ganne and Feng 2017; Hole and Natland 2020; Palin et al. 2020). However mantle temperature through time may be characterized, its determination is inevitably interwoven with other contentious geological questions, viz. (1) the relative importance of plumes and plates in mantle convection (for example, Foulger 2012; Hamilton 2019), and the significance of large mantle heterogeneities (Baumgardner 2003; Heron and Garnero 2019); and (2) the timing of the onset of plate tectonics. These issues are also important for a biblically-based understanding of mantle history, especially in the context of Flood modelling. As noted in the Introduction, Appendices 1 and 2 respectively summarize these issues and their history as recorded in some of the conventional literature. Here we consider the question of mantle thermal history and offer an assessment of this thermal history from a biblical perspective.
An indication of upper mantle temperature can be gleaned from the thickness of oceanic crust, since a higher mantle temperature is understood to imply a greater degree of decompression melting of magma upwelling beneath mid-ocean ridges, resulting in thicker crust (Klein and Langmuir 1987; McKenzie and Bickle 1988; Van Avendonk et al. 2017). Generally, once sediment cover has been discounted, this thickness is approximately 6–7 km and varies little between one ocean basin and another or with distance (treated in the literature as a proxy for age) from the spreading center, at least for full spreading rates down to 20 mm/year (Bown and White 1994; Chen 1992; McClain and Attalah 1986; White et al. 2001). However, a more recent study of oceanic crust formed since the mid-Jurassic found a statistically significant decrease in thickness through that time, implying measurable mantle cooling (Van Avendonk et al. 2017). Specifically, the average thickness of oceanic crust formed in the mid-Jurassic, associated with the breakup of Pangaea, is 1.7 km greater than the average today, a result interpreted by Van Avendonk at al. (2017) as signifying a cooling of the upper mantle by 15–20°C per 100 Ma over this period. However this thickness/time dependence is much weaker in the Pacific ocean than in the Atlantic and Indian oceans, which Van Avendonk et al. (2017) suggest is related to the origin of the ocean basins, noting that the Pacific is mostly surrounded by subduction zones, while the Atlantic and Indian oceans formed by continental rifting.
Since ocean crust thickness depends only weakly on upper mantle temperature and is subject to complicating factors (for example, spreading rate), the more usual way of assessing mantle temperature is through the record embodied in the structure and petrology of igneous and metamorphic ocean floor rocks, which can be used to assess magma source and emplacement conditions including temperature. This leads to the concept of mantle potential temperature, which is considered next.
Mantle potential temperature
In addition to temperature variations associated with mantle heterogeneities, mantle temperature in general increases with depth because of the outward flow of heat from the earth’s core. Furthermore upward movement of material in mantle convection implies decreasing pressure and hence cooling by decompression. Therefore, a useful, notionally depth-independent measure of temperature is potential temperature Tp, defined as the temperature a mantle rock sample (usually peridotite) would reach if brought adiabatically to the surface, where the pressure is atmospheric, without partial melting. If the potential temperature is above the solidus (the temperature below which the magma is completely solid) for the relevant magma composition, partial melting will occur (for example, Asimow 2017; Dalton, Langmuir, and Gale 2014; Herzberg 2011; McKenzie and Bickle 1988; Sarafian et al. 2017), in which case the eruption temperature will be lower than the potential temperature because of the latent heat (enthalpy of melting) required; this effect can be seen, for example in fig. 2 of Hole and Natland (2020, 3).
Potential temperatures cannot be measured directly, but have to be inferred from the available geochemical and geophysical evidence. This is far from simple, and a wide range of estimates based on a range of different assumptions and methods have been published. Foulger (2012), for example, lists a number of published Tp estimates ranging from 1243°C to 1688°C. These figures are obtained by a variety of methods, though Foulger (2012) describes most of them as “olivine control lines,” that is, based on lines in pressure-temperature space (for example, adiabat, dry solidus, liquidus, MgO isopleth etc), usually indexed by olivine composition. Hole and Natland (2020), who also list several published Tp estimates, define the ambient Tp as the mantle potential temperature which will produce mid-ocean ridge basalt (MORB) at spreading ridges, noting that the adiabat for convecting mantle has a slope of ~0.5°C/km. In common with many others they assume Tp~1350°C, but also note that there is a wide variation in estimates of ambient Tp, for example, 1280°C (McKenzie and Bickle 1988), 1430°C (Green et al. 2001),2 1454±81°C (Putirka et al. 2007) and 1500°C (based on an “assumed” adiabat, Presnall and Gudfinnsson 2011, fig. 2b). Putirka et al. (2007) list a whole range of earlier estimates of mid-ocean ridge Tp values, which are generally higher than the 1280°C of McKenzie and Bickle (1988), and make their own estimates on the basis of olivine-liquid equilibria; their method requires specification of five parameters including pressure and oxygen fugacity. For their own investigation of the North Atlantic Igneous Province, Hole and Natland (2020) give a figure of 1350°C based on the PRIMELT3 software (Herzberg and Asimow 2015).
Olivine is a key mineral in all methods of estimating Tp because of its high crystallization temperature, which means that it is generally the first, or one of the first, minerals to crystallize from basalts of quite different compositions. Foundational experimental work in the estimation of magma source temperatures was undertaken by Roeder and Emslie (1970), who determined the equilibria between olivine and basaltic melt at atmospheric pressure as a function of temperature through the range 1150–1300°C, with oxygen fugacity between 10–12 and 10–0.68 bar. Their results provided a basis for testing natural olivine-liquid pairs for equilibrium and fractional crystallization in basaltic magmas. Subsequent experiments by Ford et al. (1983) on cation partition coefficients in olivine-liquid systems, specifically Mg, Fe2++, Ca, and Mn in dry basic and ultrabasic magmas, demonstrated that the Mg and Fe2++ equations could be used as geothermometers. Herzberg and O’Hara (2002) used experimental data in the pressure range 0.2–7.0 GPa to calibrate models of magma source composition to infer the MgO content and potential temperature of primary magmas corresponding to peridotites from Gorgona, Hawaii, Baffin Island, and West Greenland. This work was extended by Herzberg (2004) to deal with peridotite samples from a range of sea floor locations including oceanic ridges and subduction zones, the mantle section of ophiolites, massif peridotites, and xenoliths of cratonic mantle in kimberlite. Thus, the work of Herzberg and O’Hara (2002) and Herzberg (2004) produced another olivine-based geothermometer, which became the basis of the PRIMELT software (Herzberg et al. 2007), now widely used to estimate potential temperatures. Putirka (2005) developed another geothermometer based on olivine-liquid equilibria to investigate potential temperatures at Hawaii, Iceland, and several locations on the mid-ocean ridge system.
A comparative study of these three dry geothermometers at 0.1 mPa and 15 GPa by Falloon et al. (2007) found that the Ford et al. (1983) method was the most successful in terms of reproducing experimental temperatures and olivine-melt partition coefficients. However the most widely-used of the above methods is PRIMELT, which provides ready-to-use software for estimating magma source conditions. PRIMELT1, which runs in Microsoft Excel©, was formally introduced and made generally available by Herzberg et al. (2007). It is a mass-balance method that only requires as input a primitive lava composition representative of a set of lavas containing olivine-only phenocrysts; its output is expressed in terms of melt fraction. A particular drawback noted by Herzberg et al. (2007) is that PRIMELT1 will give MgO contents 2% too high if the residue consists of garnet peridotite, as in peridotite-source melts from Hawaii. An updated version, PRIMELT2, was introduced by Herzberg and Asimow (2008). This includes garnet peridotite melting and detects complexities that can lead to overestimates in Tp by >100°C, viz. variations in source lithology, source volatile content, source oxidation state, and clinopyroxene fractionation. PRIMELT3 (Herzberg and Asimow 2015) includes further developments, notably an analysis of uncertainties in mantle potential temperature and olivine liquidus temperature. The PRIMELT3 equation for potential temperature in °C, given by the intersection in pressure-temperature space of the solid-state adiabat with the dry peridotite solidus when the MgO content in the melt is in the range 10–38wt%, is (Herzberg and Asimow 2015):
The cited Tp uncertainty is within ±42°C for variations within ±1.5wt% of MgO isopleths for partial melts of mantle peridotite; Herzberg and Asimow (2015) state that existing adiabatic melting models satisfy this constraint on melt composition.
An alternative approach to magma temperature measurement, known as “Al-in-olivine/spinel” thermometry, was pioneered by Wan, Coogan, and Canil (2008), and developed further by Coogan, Saunders, and Wilson (2014). This method is based on the temperature dependence of Al exchange between olivine and Cr-spinel, allowing the maximum temperature of coprecipitation of these phases to be determined from analysis of the compositions of equilibrium olivine-spinel pairs from primitive lavas. Its particular appeal is that, in contrast with the olivine-liquid method of Putirka et al. (2007), it is largely independent of crystallization pressure, oxygen fugacity, and melt composition, notably with respect to water and carbon dioxide content (Geng et al. 2019). In general it will produce lower estimates of potential temperature than the more traditional olivine-melt equilibrium methods (Matthews, Shorttle, and Maclennan 2016). From their calibration exercise Coogan, Saunders, and Wilson (2014) claim reproducibility of this thermometer within ±25°C. Yet another method of mantle temperature estimation was presented by Mallmann and O’Neill (2013) based on the temperature-sensitive partitioning of trace elements (specifically Sc, V, and Y) between olivine and silicate melt; the claimed accuracy of estimates based on their calibration is ±15°C. This last method (Mallmann and O’Neill 2013) was used alongside the Al-in-olivine thermometer (Coogan, Saunders, and Wilson 2014) and the olivine-equilibrium method of Ford et al. (1983) by Korneeva et al. (2020) in their compositional study of sulphide globules in olivine phenocrysts in MORB-related picrites from Kamchatka, Russia. All three geothermometers gave very similar crystallization temperatures corresponding to typical MORB melts.
All the above figures apply to relatively recent geological history, that is, since the early Cenozoic; in the original version of CPT (Austin et al. 1994) this corresponds roughly to the post-Flood period. Among the most comprehensive studies of mantle potential temperature through time, going back to the Archean, are those of Herzberg, Condie, and Korenaga (2010) and Ganne and Feng (2017). Herzberg, Condie, and Korenaga (2010), using the PRIMELT2 software (Herzberg and Asimow 2008), conclude that non-arc basalts of Archean and Proterozoic age have model primary magmas exhibiting mantle potential temperatures Tp increasing from 1350°C today to a maximum of ~1500–1600°C at 2.5–3.0 Ga, that is, decreasing through earth history: the trend converges smoothly to that of the present-day MORB source. They infer that: (1) the non-arc basalts were formed by the melting of hot ambient mantle, not mantle plumes, and that they can constrain the thermal history of the earth; (2) the mantle was warming in deep Archean–Hadean time because internal heating exceeded surface heat loss, and it has been cooling from 2.5 to 3.0 Ga to the present; (3) komatiite parental magmas reveal Tp values higher than those of non-arc basalts, which is consistent with the hot plume model, but they account for little total magmatism; (4) primary basaltic partial melts having 10–13% MgO are a feature of Phanerozoic magmatism, not of the early earth. Fig. 2 here reproduces fig. 1 in Herzberg, Condie, and Korenaga (2010), showing their results for Tp through time; note the considerable range of variation at any chosen point in geological history.
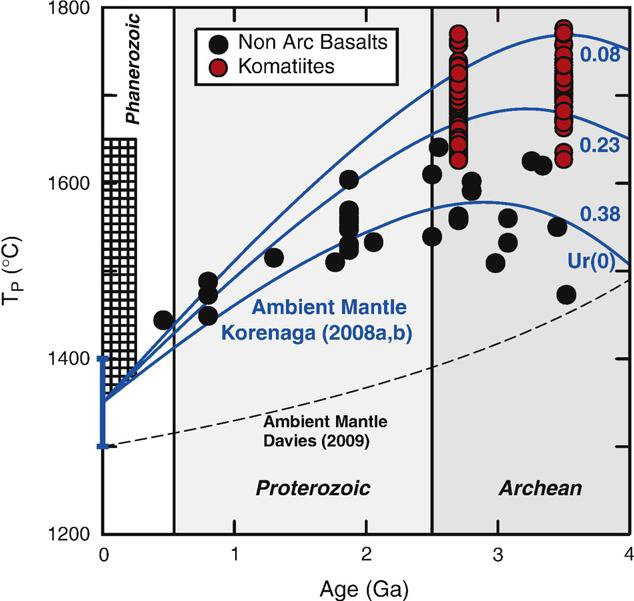
Fig. 2. Reproduction of fig. 1 in Herzberg, Condie, and Korenaga (2010), showing secular thermal earth models for ambient mantle (blue curves) compared with petrological estimates of mantle potential temperature (Tp) for non-arc lavas and komatiites with the conventional ages indicated. Numbers next to the curves are the model present-day Urey ratio values (Korenaga 2008a; 2008b; see also geological glossary). Tp values are based on the equation Tp(°C) = 1463 + 12.74*MgO–2924/MgO (Herzberg et al. 2007; Herzberg and Asimow 2008) where MgO is the primary magma MgO content in wt%. Gridded region depicts the range of Tp for Phanerozoic plume-related magmas, which is typically of order 100°C.
Using PRIMELT3 Ganne and Feng (2017) investigated potential temperatures in more than 22,000 samples of mafic and ultramafic rocks (which have high proportions of magnesium and iron and SiO2 content between 43 and 51 wt%) from the GEOROC database, which was inspired by the pioneering work of Keller and Schoene (2012). Ganne and Feng (2017) filtered out intrusives (that is, plutonic rocks such as gabbro, diorite etc) and to avoid selection bias did not preselect into different trace element ranges. In contrast to Herzberg, Condie, and Korenaga (2010) they did not filter out arc basalts from the database. The acknowledged danger in this is that PRIMELT has been calibrated using data on dry materials, whereas arc basalts are produced in continental margins and subduction zones where there are usually significant proportions of water and other volatiles. However their results and overall conclusions were similar to those of Herzberg, Condie, and Korenaga (2010); see figs. 3a–c, which are based on figs. 7–9 respectively of Ganne and Feng (2017). These relate to different assumed redox conditions in the magma source, the most reducing conditions being represented in fig. 3a, the most oxidizing in fig. 3c, and intermediate conditions (as favoured by Ganne and Feng), in fig. 3b. Ganne and Feng (2017) interpret the range of Tp values at any one point in time as representing a mix of melting products of ambient mantle (Tp minima) and plumes (Tp maxima), and note that the difference between these Tp extrema, ~170°C, is practically the same throughout earth history. They also acknowledge that their reported Tp values depend on the assumed redox conditions in the magma source. As for the long-term trend, Ganne and Feng (2017) cite a cooling of ~150°C in the mantle average potential temperature between 2.5 Ga and the present day, that is, at the lower end of the range found by Herzberg, Condie, and Korenaga (2010). Ganne and Feng (2017) deduce an average Tp of 1350°C for the present-day mantle source of MORBs. Despite the caveats noted above, this work (Ganne and Feng 2017) probably represents the most reliable representation of mantle temperature currently available.
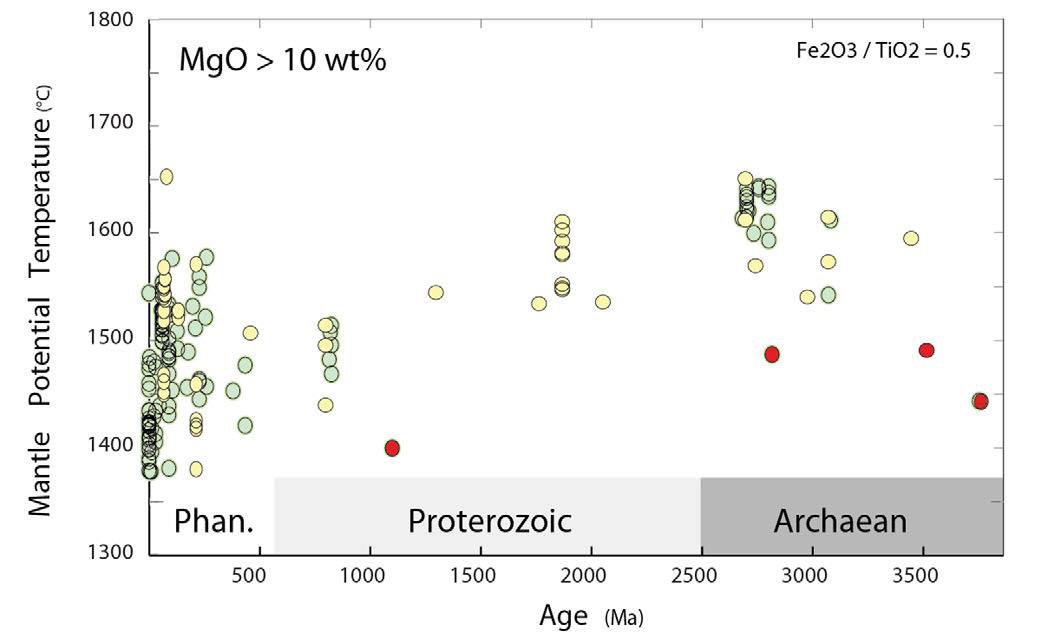
Fig. 3a. Reproduction of fig. 7a in Ganne and Feng (2017). Mantle potential temperatures (Tp) plotted here are calculated with reduced conditions (Fe2O3/TiO2 = 0.5) in the source and filtered with MgO < 10 wt% (green dots). Samples gathered by Herzberg and coworkers (Herzberg and Gazel 2009; Herzberg, Condie, and Korenaga 2010) on plume-like and MORB-like basalts have been reanalyzed using PRIMELT for this plot (yellow dots). Red dots indicate low Tp values considered suspect by Ganne and Feng (2017, fig. 7 caption) as “possibly corresponding to more evolved liquids where pyroxenes fractionated.”
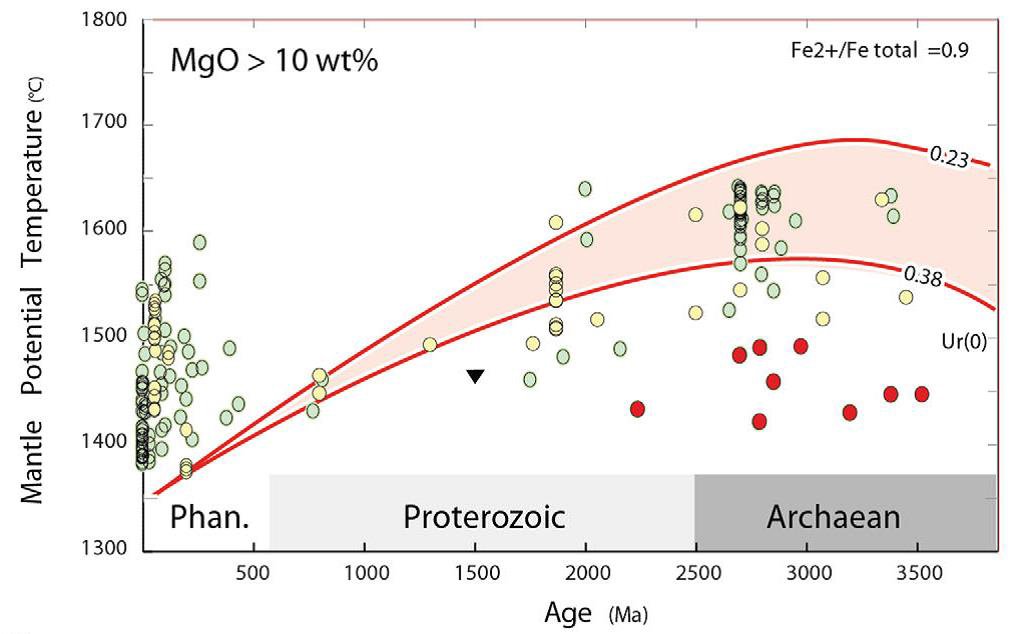
Fig. 3b. Reproduction of fig. 8a in Ganne and Feng (2017). Mantle potential temperatures (Tp) plotted here are depicted from the composition of primary magmas assuming intermediate redox conditions (FeO/FeOtotal = 0.9). Green and yellow dots correspond to GEOROC samples and samples gathered by Herzberg and coworkers (Herzberg and Gazel 2009; Herzberg, Condie, and Korenaga 2010) respectively. Red dots represent suspect Tp values. The number next to each red curve is the model present-day Urey ratio (Ur), defined as the internal heat production for the entire earth divided by total surface heat flow.
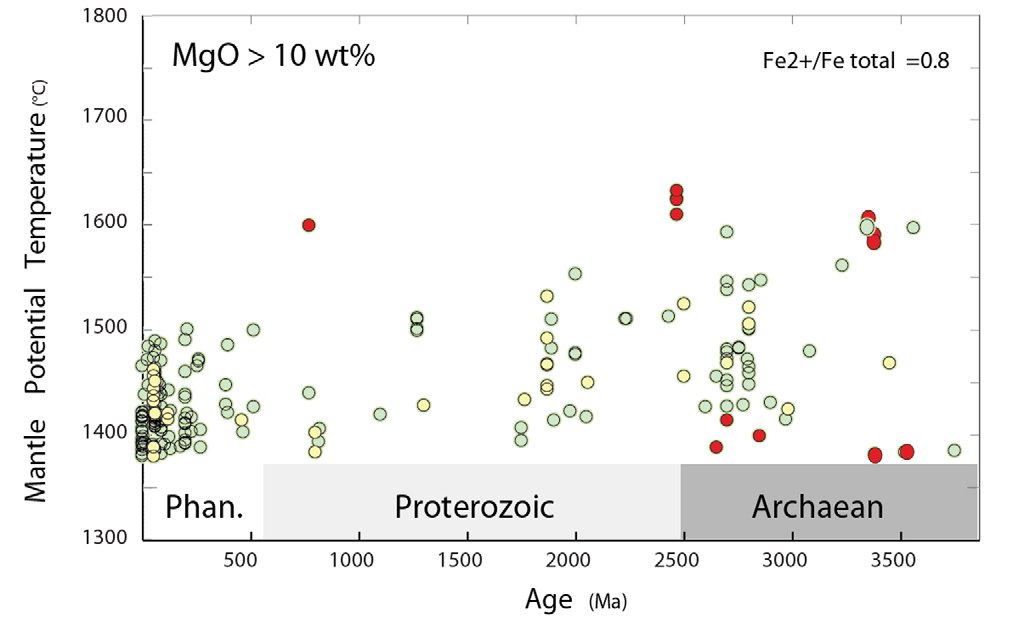
Fig. 3c. Reproduction of fig. 9a in Ganne and Feng (2017). Mantle potential temperatures (Tp) plotted here are depicted from the composition of primary magmas assuming fixed redox conditions (FeO/FeOtotal = 0.8). Green and yellow dots correspond to GEOROC samples and samples gathered by Herzberg and coworkers (Herzberg and Gazel 2009; Herzberg, Condie, and Korenaga 2010) respectively. Red dots represent suspect Tp values.
The above figures for mantle temperatures through time largely refer to the mantle beneath the oceanic crust, and do not fully reflect the effect of continental crust which, being thicker than oceanic crust, has an insulating effect, which in turn leads to higher temperatures, at least in the upper mantle (Coltice et al. 2007; 2009; Doblas et al. 2002; Rolf, Coltice, and Tackley 2012). The effect is greatest for supercontinents, which tend to induce very long-wavelength mantle convection and large lateral temperature differences within the mantle. These may in turn destabilize the supercontinents, thus contributing to the supercontinent cycle (Lenardic et al. 2011; Lenardic 2017; Phillips and Coltice 2010).
To summarize: (1) the average mantle potential temperature has fallen by approximately 150°C since the lower Paleoproterozoic—there is no obvious marker in the geological record of major global heating or cooling events corresponding to critical points within a CPT-style Flood scenario; (2) the difference between the mantle Tp extrema at any one point in earth history has remained practically the same (~170°C) throughout; (3) these figures do not include any sampling of sub-continental mantle temperatures and are probably not fully representative of the whole mantle. However, points (1) and (2) assume that the temporal ordering of the data points used by Herzberg, Condie, and Korenaga (2010) and by Ganne and Feng (2017) is essentially correct despite the uniformitarian timescale for earth history being massively inflated. The lithostratigraphic geological column, that is, as described in terms of sequences, groups, formations, and beds, is broadly accepted by creation geologists (Snelling et al. 1996), but its interpretation as chronostratigraphic, that is, in terms of era, period, epoch, and age, and the associated terms late, middle and early, is rejected. A systematic reanalysis from this perspective of the provenance of the rock samples investigated by Herzberg, Condie, and Korenaga (2010) and by Ganne and Feng (2017) and their stratigraphic relationships (where these can be ascertained) would be needed to clarify whether the published temperature trends are meaningful. Short of this enormously laborious exercise, which seems highly unlikely to prove fruitful, the findings of these authors should be taken as reliable. On point (3), little directly relevant data is available.
Heat Deposited in the Formation of Ocean Floors
This cooling problem was addressed on the ocean-basin scale through simple spreadsheet-based calculations presented at the 2018 International Conference on Creationism (Worraker and Ward 2018). The magnitude of the problem in terms of the quantity of heat deposited is fixed by the ocean floor area in question, the assumed oceanic lithosphere thickness and the thermophysical properties of the cooling, solidifying magma. Since Worraker and Ward (2018) only considered the present-day ocean floors, this estimate does not include heat deposited in the oceans and atmosphere by material which erupted, cooled and was then subducted before the end of the Flood, nor, as noted in the Introduction, does it include any contribution due to AND (Accelerated Nuclear Decay). It therefore represents a lower limit on the total heat deposition during the Flood. Nevertheless this heat, if transferred adiabatically to the oceans, would be overwhelming, more than 30 times sufficient to boil off all of earth’s ocean water. The fundamental result of the modelling exercise undertaken by Worraker and Ward (2018), in which a variety of artificial heat sinks within the cooling magma were invoked, was that observed bathymetry and surface heat flow data could not be reproduced within their model framework. In that paper it was deemed inappropriate to otherwise modify the thermophysical properties of the cooling magma (notably thermal conductivity, specific heat, density, and thermal expansion coefficient), as these are all closely interrelated at the fundamental atomic and molecular level; the evidence for AND deduced from the results of the RATE project is essentially confined to nuclear processes (Vardiman et al. 2005).
By way of follow up, we now consider: (i) the effect of variations in the parameters of the model used by Worraker and Ward (2018); (ii) the possibility of thermal radiation in a fourth spatial dimension as the means of rapid cooling; (iii) what else can be deduced about the cooling process, including the time taken, from the available field evidence. Diffusion chronometry, which is important with regard to cooling timescales, an aspect of point (iii) here, is introduced in Appendix 4.
Model variations
Key input parameters to the model used by Worraker and Ward (2018) are the depth L and thermal conductivity k of the cooling magma which now constitutes the earth’s oceanic lithosphere. The thermal conductivity of silicate minerals, which are essentially electronic insulators, is the sum of two transport mechanisms: lattice (or phonon) transport and radiative (or photon) transport. Grose and Afonso (2019) propose models for the radiative component krad for the upper mantle, giving an estimate of about 2.0–3.5 Wm−1K−1 for a representative mean grain size in the range 0.01–1 cm; this translates to a total thermal conductivity in the range 5.5–7.0 Wm−1K−1, approximately twice the value used by Hasterok (2013), Parsons and Sclater (1977), and Stein and Stein (1992). However Grose and Afonso (2019) make clear that krad varies considerably as a function of mineral composition, grain size and temperature, and thus with both depth and distance from the spreading axis. In the uniformitarian models cited above the cooling magma has been treated as equivalent to present-day oceanic lithosphere, with depth ranging from 90 km to 125 km. Thus the results of further simulations embodying different values of magma depth L and values of k varying with position and temperature would be of some interest. Insights may be gained by using considerably wider ranges of L, perhaps down to 10 km or 5 km (roughly corresponding to the average thickness of oceanic crust), to accommodate the idea that the magma which solidified to form the crust was emplaced independently of the underlying upper mantle material, and k, perhaps up to 10 or 100 Wm−1K−1 or more, i.e. in both cases beyond the ranges considered plausible in normal conditions. Given that Flood conditions were anything but normal this would seem a reasonable approach to gleaning evidence relevant to ocean floor cooling. However, the discrepancy between the observed magnitude and pattern of ocean floor heat fluxes and bathymetry and those predicted by Worraker and Ward (2018) is so great that such an exercise seems unlikely to produce a satisfactory solution.
We also ask whether significant cooling could have been accomplished by the upward convection of a large quantity of heat from the spreading centers by supersonic steam jets carrying entrained ocean water, alias the “fountains of the great deep” of Genesis 7:11 and 8:2. Although this phenomenon was contemplated by Baumgardner (2003) as a possible mechanism for rapid heat removal, he did not pursue the idea further because its cooling potential was too limited. A similar, even bolder idea is proposed in Walt Brown’s hydroplate theory (Brown 2020), which contemplates hypersonic jets of water driven upwards at 150 times the speed of sound by the release of supercritically pressurized subterranean water at the initial breaking open of the mid-ocean ridges corresponding to Genesis 7:11. However an earlier version of this theory—no different from the 2020 version with regard to this feature—was critiqued by Oard (2013) and the astronomical aspects in particular by Faulkner (2013), who showed that the hypersonic jets would have produced an intolerable level of heating of earth’s atmosphere. There is no obvious way of developing Brown’s ideas further to help explain ocean floor cooling.
Cooling by 4D thermal radiation
In a paper also presented at the 2018 International Conference on Creationism, Humphreys (2018) proposed a mechanism based on thermal radiation in a fourth spatial dimension for rapid removal of excess radiogenic heat during the Flood (Humphreys 2000; 2005), suggesting that this would also provide rapid cooling of batholiths and new ocean floors as they formed. Humphreys’ proposal is based on his singular interpretation of Genesis 7:11 and 8:2, which speak respectively of the opening and closing of the “windows of heaven.” Humphreys views this as the opening and subsequent closing of the fourth spatial dimension in 4D hyperspace.3 The idea is that the fourth dimension, which is normally unobservable because extremely thin (rolled-up), was opened during the Flood, allowing the emission of thermal (or blackbody) radiation from all the atoms in a volume rather than simply from the surface, thus enabling far more efficient cooling than would be obtained by the normal mechanisms of conduction and surface radiation. Humphreys (2018) treats the total heat loss by radiation as proportional to the fourth power of absolute temperature as in the familiar 3D case; he does not attempt a formal derivation of the temperature dependence or of the constant of proportionality.
There are several reasons, however, why Humphreys’ (2018) proposal cannot provide a solution to the problem of cooling the ocean floors. First, thermodynamic considerations imply that the rate of blackbody radiation depends on the number of spatial dimensions (Alnes, Ravndal, and Wehus 2007; Cardoso and Castro 2005). The total blackbody radiation emitted by an (N-1)-dimensional surface (or hypersurface) in an N-dimensional space (or hyperspace) can be expressed in the form
where RT is the blackbody radiance at absolute temperature T, ε is emissivity, and σN is the generalized Stefan-Boltzmann constant for N dimensions. For N = 4, corresponding to 4D hyperspace, (2) becomes
where σ4 = 3.02064572 × 10–5 Wm-3K–5; Appendix 3 gives details of the supporting calculations.
Consider the application of these formulae to heat loss by radiation from (1) the human body, and (2) cooling magma (cf. Humphreys 2018). For the purpose of illustration and discussion we use very rough, simplistic estimates of the necessary parameters.
In case (1), in 3D, we follow the reasoning of Humphreys (2018) regarding the heat balance for humans on the Ark, that is, we assume a body mass of (say) 70 kg, and hence a volume of ≈ 0.07 m3, and a 24-hour average heat production of 100 W, corresponding to an effective metabolic rate of ≈ 2100 kcal/day. Humphreys (2018) suggests that a 10% increase in these rates would be easily manageable. Assuming core body temperatures of 37°C (310 K), the total radiation heat loss in 4D hyperspace would be 8.65 × 107 W/m3, or 6.05 × 106 W. As it stands this is vastly greater than could be sustained naturally; even if reduced by three orders of magnitude, it would almost instantly reduce the subject’s core body temperature to the environmental temperature, resulting in a quick death. However, Humphreys (2018) includes an opening angle θc for radiation into the fourth dimension, essentially representing the effect of an extremely high speed of light in that direction, and employs θc to represent emissivity in the 4D case. The necessary value of θc cited by Humphreys (2018) to reduce the radiation heat loss from an average human to a manageable level is 0.295 milliradian; the same reasoning applied to equation (3) here, ignoring any incoming radiation, would require ε = 1.652 × 10–6, corresponding to θc = 11.8 milliradian, or approximately 0.677°. This in turn implies that the light speed in the fourth dimension is more than 80 times the canonical speed of light.
In case (2), assuming a magma eruption temperature of 1500 K (as suggested by Humphreys 2018), the radiation heat loss through 100 km depth (corresponding to the approximate thickness of the oceanic lithosphere) in 4D would be 2.3 × 1011 Wm–3, or 2.3 × 1016 Wm–2 in terms of ocean floor area; these figures assume the canonical value of the speed of light in each dimension. Since the total heat deposited in forming new oceanic lithosphere is 3.9 × 1014 Jm–2 (Furlong and Chapman 2013), the timescale for the whole cooling process, even allowing for a declining level of radiation as the magma temperature falls, is very short: cooling is complete within 0.4 seconds. Appendix 3 gives the details of the calculation. In the case of a very high speed of light in the fourth dimension as suggested above, corresponding to emissivity ε = 1.652 × 10–6, this cooling time increases to 1.9 days, still extremely short in comparison with any natural diffusion-controlled cooling timescale.
Humphreys’ (2018) assumption of a very high speed of light in the fourth spatial dimension constitutes a major inconsistency in employing the theory presented by Alnes, Ravndal, and Wehus (2007) and by Cardoso and de Castro (2005), who assume the same speed of light in all spatial directions. Given that Humphreys’ (2018) use of a very high speed of light in the fourth spatial dimension is not essential to the idea of volume cooling by radiation in 4D (Humphreys 2022), it seems that there are two ways to apply his idea to the cooling of newly-forming ocean floor during the Flood: (i) applying the formal theory developed by Alnes, Ravndal, and Wehus (2007) and by Cardoso and de Castro (2005) as it stands and presenting the human and geological consequences—these would seem to be so drastic that this scenario is unsupportable; (ii) undertaking a new development of the equivalent theory in the case of a very high speed of light in one dimension. In the latter case, it would need to be demonstrated how Maxwell’s equations, Planck’s constant, the Boltzmann constant, etc., should be generalized self-consistently. How, and even if this could be done, is not clear at the present time. However, an even more fundamental problem arises at the atomic scale, since in 4D space electron orbits within atoms would be unstable, implying that stable atoms could not exist (Barrow and Tipler 1986, 265; Rees 1999, 149–151); this problem alone looks like a show-stopper!
Humphreys’ (2018) proposal of ocean floor cooling by radiation into a fourth spatial dimension may be seen as an attempt to bypass the natural process of upward heat conduction in order to facilitate rapid removal of the heat deposited by the cooling, solidifying magma. The effect of such radiation cooling can be represented by including appropriately tailored heat sinks in calculations of the kind undertaken by Worraker and Ward (2018); note that this tailoring must include a weighting which favors radiation from shallower levels as against deeper levels within the earth, since the T5 dependence of this radiation strongly favors the hotter (deeper) levels. In the cases investigated there, no such heat sink formulation was found which could duplicate the observed patterns of bathymetry and heat flux. This result was explained by Worraker and Ward (2018) in terms of an unavoidable near-surface thermal boundary layer at early times. An alternative view is to recognize that the bathymetry or surface subsidence represents the integrated heat loss through a section of the lithosphere at a particular distance from the spreading center, corresponding to a particular elapsed time since eruption, while surface heat flux indicates the present-day vertical temperature gradient immediately adjacent to the surface, remembering that subsidence increases with time and heat fluxes decrease with time. Uniformitarian models (for example, Hasterok 2013; Parsons and Sclater 1977; Stein and Stein 1992), which do not introduce arbitrary heat sinks, reproduce the observed patterns of these variables to a fair degree of accuracy. Not surprisingly, this natural relationship between bathymetry and surface heat flux would almost certainly be disrupted by the introduction of arbitrary heat sinks and hence cannot be reproduced when 4D thermal radiation is proposed as the dominant cooling mechanism.
A further aspect of Humphreys’ (2018) proposal is that 4D radiation cooling could only operate in the interval between Genesis 7:11 and 8:2, that is, the first 150 days of the Flood proper, whereas the Flood as a whole lasted 371 days up to the day Noah and all on the ark disembarked (Genesis 8:18–19; see Barrick 2008).4 Thus, unless widespread vigorous volcanism in the ocean basins stopped suddenly on day 150 of the Flood and never recurred, another hitherto unrecognized mechanism must have replaced the 4D thermal radiation thereafter until present-day levels of volcanism prevailed; geologically, this appears highly unlikely. Even if the Flood proper did not end until the deposition of Pliocene rocks had begun, geological activity, including volcanism, seems to have occurred throughout the Phanerozoic in the form of ocean floor spreading, the eruption of Large Igneous Provinces and, as seen today, around the Pacific Rim and at mid-ocean ridges. Further discussion of this point seems redundant here since there are enough insurmountable problems with the idea of 4D radiation cooling to discount it as a realistic possibility. Furthermore Humphreys’ interpretation of the opening of the “windows of heaven” as referring to the temporary opening of a fourth spatial dimension, although formally legitimate, is far from obvious in the water-saturated context of the biblical description of the Flood.
Field evidence
What else can be learned about the pattern and mode of cooling and related timescales from the evidence of the rocks in the ocean floors themselves? The conventional picture of timescales associated with seafloor spreading and the formation of the ocean basins is based on seafloor isochrons reconstructed from magnetic data and fracture zone identifications; the isochrons themselves are calibrated using radioisotope data (Cande and Kent 1995; Gradstein, Ogg, and Hilgen 2012; Müller et al. 1997, 2008; Seton et al. 2012). We have already noted that uniformitarian models of ocean floor cooling (for example, Hasterok 2013; Parsons and Sclater 1977; Stein and Stein 1992) reproduce the observed patterns of bathymetry and surface heat flux quite well. Note also that this measure of validation on the large ocean-basin scale, although presented in terms of the global uniformitarian timescale, is genuine, and in this case is not due merely to circular reasoning. However, Dalton, Wilson, and Herbert (2022) have reported a slowdown of 35% in the global production rate of oceanic crust over the last 15 Ma in the conventional geological timescale, which was attributed mainly to a general reduction in plate speeds. We ask whether this could represent the final stage of deceleration from the high-speed peak (~1 ms–1 or more) implied by CPT models of the Flood (Baumgardner 2003; Cho et al. 2018)? Investigation of this question by creation scientists may prove to be fruitful.
We now return to consider the pattern and mode of cooling and related timescales in the formation of the ocean floors. Recent investigations of the lowermost oceanic crust at the fast-spreading East Pacific Rise have concluded that it cooled relatively slowly, that is, largely by upward heat conduction rather than by hydrothermal activity near the ridge (Faak, Coogan, and Chakraborty 2015; Faak and Gillis 2016; Maher et al. 2020; 2021). Maher et al. (2020; 2021) focus on the magnetic structure of the lower oceanic crust within a few kilometers of the East Pacific Rise; magnetic polarity boundaries are treated as proxies for crustal isotherms, hence constraining the form and timescales of cooling and crustal accretion. Given a crustal structure consisting of a region of dikes of order 1.5 km deep underlain by gabbro of at least the same thickness, Maher et al. (2021) found that the dike/gabbro boundary, approximately marking the 500°C isotherm, was close to horizontal for several kilometers from the spreading axis. Their results were deemed incompatible with deep hydrothermal cooling within a few kilometers of the axis, instead suggesting a broad, hot axial zone extending roughly 8 km off-axis. Faak, Coogan, and Chakraborty (2015) and Faak and Gillis (2016) investigated the thermal history of the lower oceanic crust in the vicinity of the East Pacific Rise using diffusion chronometry, specifically Mg diffusion in plagioclase (Faak, Coogan, and Chakraborty 2015) and a combination of Mg diffusion in plagioclase and Ca diffusion in olivine (Faak and Gillis 2016). Their combined results indicate very slow cooling rates in the range 0.0001–0.005°C yr–1; cooling rates decrease strongly with increasing depth. Accordingly, cooling within the plutonic complex must have taken place largely by conduction, implying that lower crust formed at fast spreading rates remains hot in the near-axis environment. This in turn indicates limited hydrothermal cooling.
A very different conclusion was reached by Sun and Lissenberg (2018a) using analysis of Mg and rare earth element (REE) bulk-diffusion between coexisting plagioclase and clinopyroxene. Analysis of their samples, taken from oceanic gabbros from the fast-spreading East Pacific Rise at Hess Deep, implied that the lower oceanic crust crystallized at temperatures of 998–1353°C with cooling rates of 0.003–10.2°C yr–1. From stratigraphic variations of estimated cooling rates and crystallization temperatures they inferred deep hydrothermal circulations and in situ solidification of various replenished magma bodies. These conclusions conflict sharply with those of Faak, Coogan, and Chakraborty (2015) and of Faak and Gillis (2016). The methodology of Sun and Lissenberg (2018a) was criticized by Faak et al. (2018) but defended by Sun and Lissenberg (2018b). At this point in time it is not clear which view is closer to reality; the controversy noted here is a reminder that extreme care in sampling, measurement, and data interpretation is necessary to obtain a clear, reliable picture of the cooling process.
Extensive analysis of gabbro samples from the Oman Ophiolite, which is thought to provide the best available analogue for modern fast-spreading oceanic crust (Phipps Morgan and Chen 1993), was undertaken by Müller et al. (2022) and by Koepke et al. (2022). From trace element systematics, REE crystallization temperatures, petrography, and petrology, these authors concluded that the lower two-thirds of the gabbroic crust were formed by sill intrusions and in situ crystallization, while the upper third is dominated by downward differentiation of a primitive parental melt that originated from an axial melt lens located at the top of the gabbroic crust.
Some ocean floor regions form from ultra-slow spreading ridges, for example, the South-West Indian Ocean Ridge, where the full spreading rate is estimated to be 14 mm/yr–1 (for example, Schwartz et al. 2009). We have previously noted that for full spreading rates down to 20 mm/year oceanic crust thickness is generally fairly constant and lies in the range 6–7 km. However, at lower spreading rates the thickness and basalt chemistry of the crust are much more variable; here the cooling process is thought to be dominated by conductive cooling of mantle material upwelling beneath the spreading ridge (Bown and White 1994; White et al. 2001). Fan, Olive, and Cannat (2021) have designed a new model for the thermal structure of ultraslow ridge axes in which episodic magma intrusions provide heat to a hydrothermal convection system confined to depths < 6 km. Their model is claimed to successfully explain the depth to the brittle-ductile transition as well as the depth of MORB crystallization at the Mid-Cayman Spreading Center (in the Caribbean) by invoking repeated emplacement of sills every
20–50 ka.
Crust formation at intermediate spreading rates, for example, at the Costa Rica Rift in the equatorial East Pacific, seems to be controlled by a delicate balance of magmatism, faulting, and fluid circulation across a range of scales, which may also be influenced by large lithosphere-scale structural and/or thermal heterogeneity (Robinson et al. 2020; Wilson et al. 2019). Clearly the question of the pattern, mode, and timescales of cooling of the material forming oceanic crust is very complex. There are no straightforward answers at present, especially as several distinct mechanisms are involved whose relative significance is linked to the spreading rate. This is a challenging but important field of research for creation scientists working in the context of Flood modelling.
Heat Deposited in the Formation of Large Igneous Provinces (LIPs)
After the ocean floors, which are formed continuously from magma emerging at mid-ocean ridges and spreading centers, LIPs represent the largest igneous structures on earth and are thus important in any geological narrative of earth history, creationary or secular. The best-known LIPs, continental flood basalts, for example, the Columbia River Basalt Group (or CRBG), the Deccan Traps and the Siberian Traps, were recognized long before the 1980s. Indeed the spectacular columnar basalt formations characteristic of many continental flood basalts were known from ancient times, and had captured the imagination of visitors and artists from before the era of modern geology (figs. 4–6). However LIPs were not treated as a special category of igneous formation until the early 1990s after the recognition, largely through the pioneering work of Coffin and Eldholm, of major igneous provinces submerged along continental margins and in ocean basins (Coffin and Eldholm 1991; 1992; 1994; Eldholm and Coffin 2000). The current generally-accepted definition of LIPs, which assumes conventional geological timescales, is given by Bryan and Ernst (2008, 175):
Large Igneous Provinces are magmatic provinces with areal extents >0.1 mkm2, igneous volumes >0.1 mkm3 and maximum lifespans of ~50 myr that have intraplate tectonic settings or geochemical affinities, and are characterized by igneous pulses(s) of short duration (~1–5 myr), during which a large proportion (>75%) of the total igneous volume has been emplaced.
Thus, in geological terms, LIPs represent enormous emplacements of magma, much larger than the products of any currently active magmatic system on earth: note that Coffin and Eldholm (1992; 1994) and Eldholm and Coffin (2000) expressly exclude provinces resulting from “normal” seafloor spreading. LIPs consist of extrusive and intrusive material, generally in the form of repeated short pulses over relatively short timescales. In continental settings, many LIPs were initially emplaced in the interiors of tectonic plates and on or along the edges of Archean cratons just before continental breakup or were emplaced into areas undergoing extension; they are now located along rifted continental margins. The same temporal relationships are also seen with many oceanic LIPs subsequently rifted apart by new spreading ridges (for example, the reconstructed Ontong Java–Manihiki–Hikurangi and Kerguelen–Broken Ridge oceanic plateaus). Where the first stage of such LIPs precedes the development of a new mid-ocean ridge system, or where it follows the cessation of seafloor spreading (for example, as in ocean basin flood basalts), these LIP events are classed tectonically as intraplate. Bryan and Ernst (2008) note that although LIPs are predominantly mafic (Mg- and Fe-rich) in composition, they can also contain significant ultramafic and silicic components, and a few are dominated by silicic magmatism—hence the recognition of Silicic Large Igneous Provinces (for example, the Sierra Madre Occidental of Mexico) as a sub-group (Bryan 2007; Bryan and Ferrari 2013).5 Bryan and Ferrari (2013) also note that since the initial recognition of LIPs was focused largely on well-preserved Mesozoic and Cenozoic formations, the key concepts were formulated in terms of these relatively young rocks (Ernst 2007), yet LIPs are found right through the geological record; in conventional terms the oldest are dated at 3.79 Ga (Isley and Abbott 1999; 2002; Ernst et al. 2013). Ernst (2007) notes the occurrence of 150 LIP events in total; Bryan and Ferrari (2013) cite a global average of one LIP event every 20 Ma, but also note that definite clusters occur at times of supercontinent breakup. Older LIPs are generally poorly preserved: Paleozoic and Proterozoic LIPs usually consist of flood basalt remnants and the exposed plumbing system represented by giant dyke swarms, sill provinces and layered intrusions (Ernst 2007). However, the plumbing systems revealed in these older provinces, which are not normally accessible in younger, better-preserved provinces, are regarded as a key source of information, especially for supercontinent reconstruction (Ernst et al. 2013).
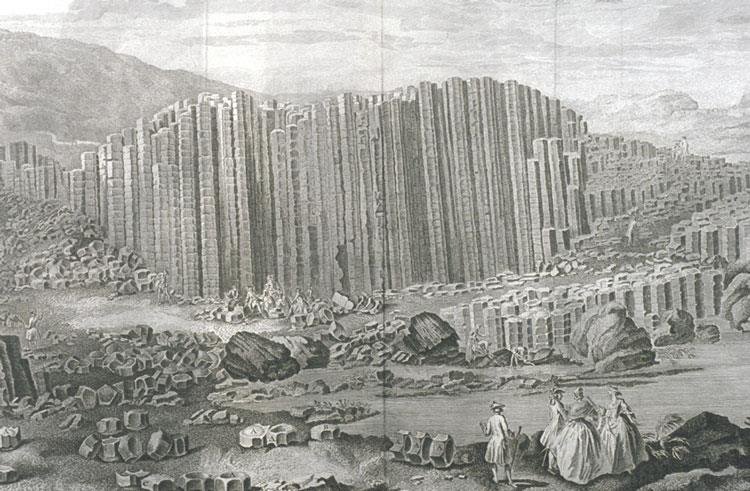
Fig. 4. Engraving of gouache painting of Giant’s Causeway, County Antrim, Northern Ireland by Irish landscape artist Susanna Drury (later Susanna Warter, 1698–c.1770). Formal title “A View of the Giant’s Causeway: East Prospect.” The Dublin Society (later the Royal Dublin Society) presented her with its first award, worth £25, in 1740 for her paintings of the Giant’s Causeway. https://commons.wikimedia.org/wiki/File:Drury_-_View_of_the_Giant%27s_Causeway.jpg.
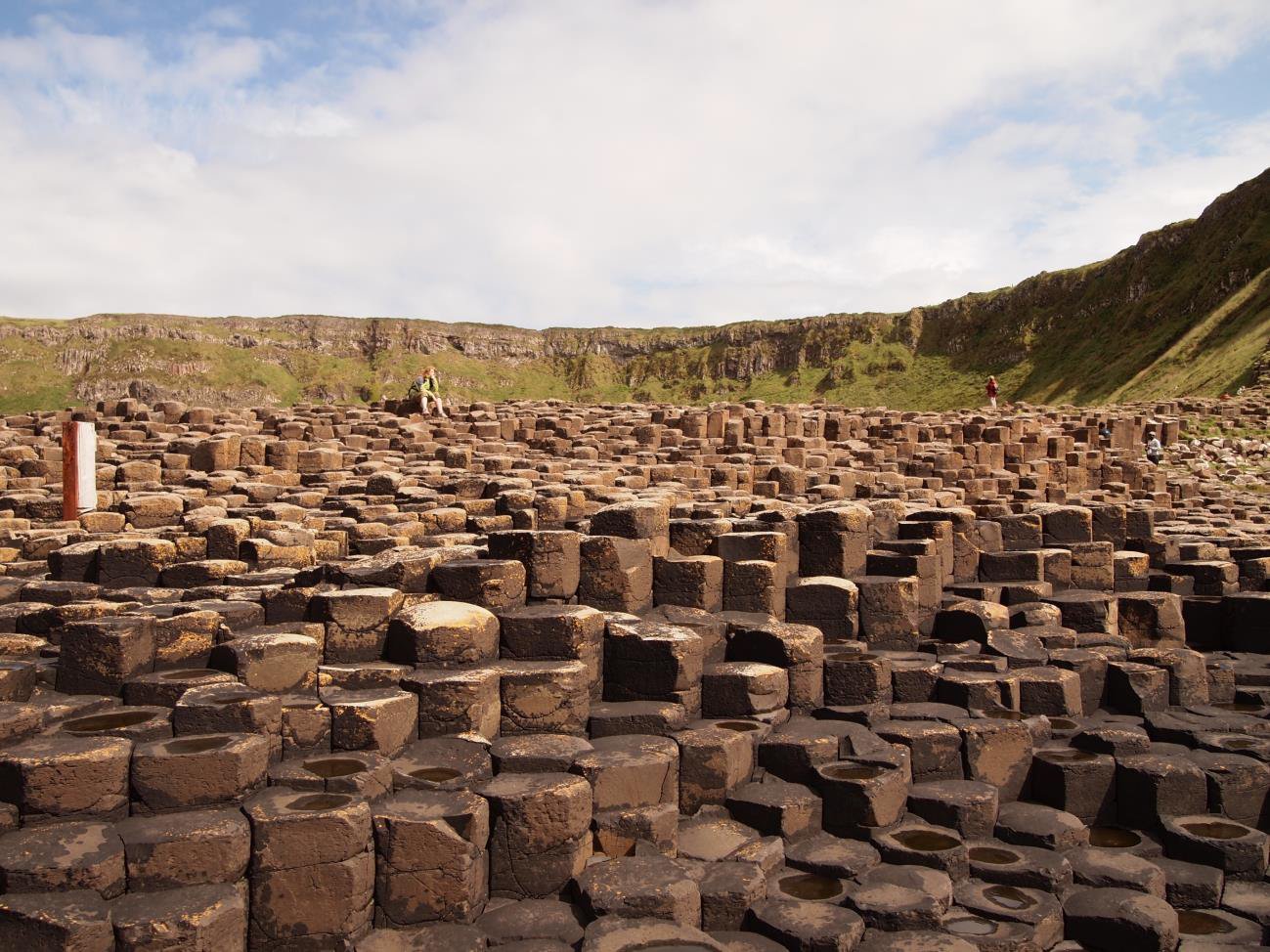
Fig. 5. Modern photograph of Giant’s Causeway, County Antrim, Northern Ireland. The people in the photograph give an idea of the scale of the basalt columns. https://commons.wikimedia.org/wiki/File:Columnar_Basalt_Giants_Causeway_Northern_Ireland.jpg.
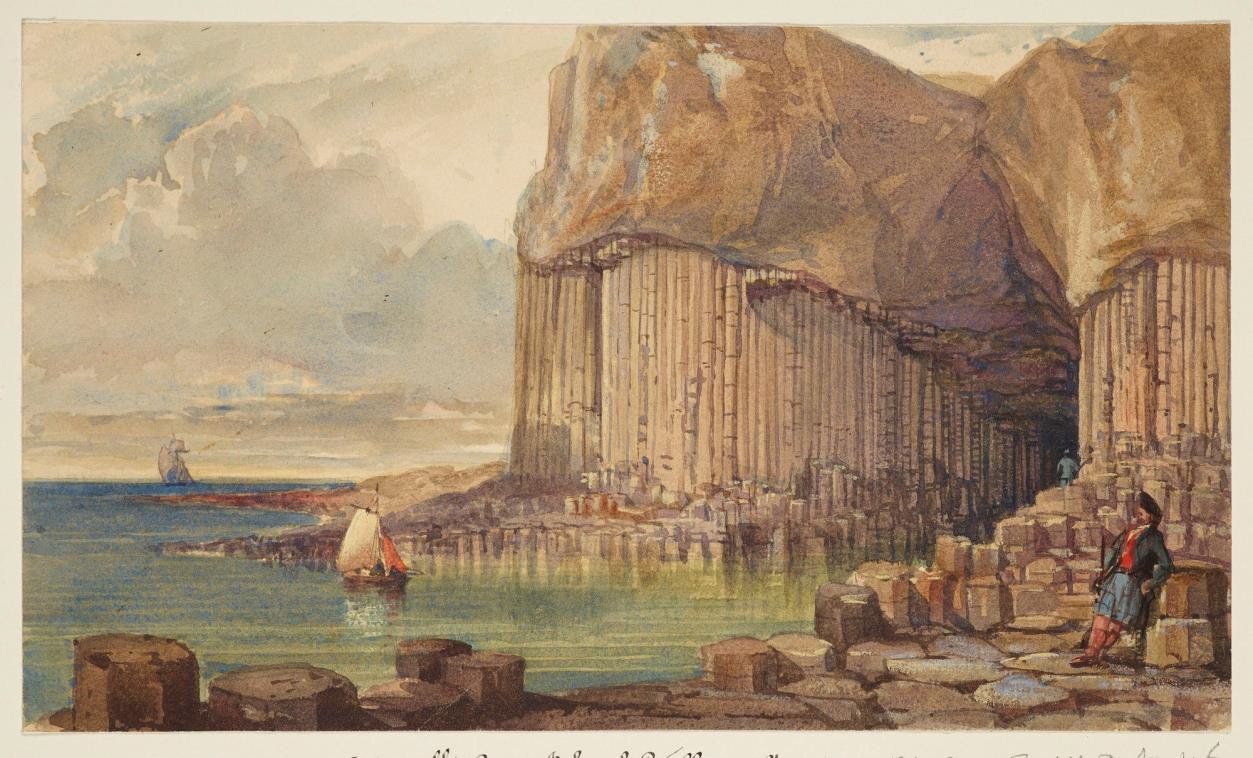
Fig. 6. Watercolour painting (c. 1847) of the entrance to Fingal’s Cave, Isle of Staffa, Hebrides, Scotland by William Leighton Leitch (1804–1883). Leitch tutored Queen Victoria and some of her children for almost 20 years in watercolour painting. The Queen had visited in August 1847 and wrote in her journal: “the wonderful Basaltic formation, which is most extraordinary...we turned the corner to go into the renowned Fingall’s Cave, the effect is splendid, like a great entrance to a hall, all vaulted but it looked rather awesome as we entered...the rocks were all pink, blue and green which had a beautiful effect under water. The sea is immensely deep inside.” https://www.rct.uk/collection/919679/fingals-cave-isle-of-staffa.
While plate tectonic theory has focused attention on plate-boundary processes to explain magmatism, the recognition of LIPs as recording major mantle melting events supposedly unrelated to normal seafloor spreading and subduction is seen as adding another dimension to the theory. LIPs have been invoked in the development of the mantle plume hypothesis to explain intraplate volcanism, including hotspots, far removed from plate boundaries (for example, Campbell 2001; Ernst and Buchan 1997; Griffiths and Campbell 1990; Morgan 1971; Richards, Duncan, and Courtillot 1989). However, the above definitions and categorizations of LIPs have not been universally accepted in the earth science community. For example, Sheth (2007) proposes a systematic hierarchical terminology for LIPs, suggesting that the term LIP should apply to any igneous province with outcrop area of at least 50,000 km2. Within this framework, the bulk of the ocean floor (in direct contrast to the definitions of Coffin and Eldholm 1992; 1994 and Bryan and Ernst 2008) qualifies as a LIP. In Sheth’s (2007) scheme provinces such as the Deccan and Whitsunday provinces should be called Large Volcanic Provinces (LVPs), whereas large intrusive provinces (mafic-ultramafic intrusions, dyke/sill swarms and granitic batholiths) should be called Large Plutonic Provinces (LPPs). Sheth (2007) proposes further subdivisions based on rock type (felsic, mafic, or ultramafic, of sub-alkaline or alkaline affinity), and geological setting (continental or oceanic). Although this system has not been generally adopted, it naturally includes granite plutons and batholiths (the largest plutons), which were investigated from a creation science perspective by Snelling and Woodmorappe (1998; 2009) and Snelling (2008), who showed how these large granitic formations could have erupted and cooled within biblically-compatible timescales. This in turn suggests the possibility that the eruption and cooling of LIPs more generally can be addressed through a similar approach to that employed by Snelling and Woodmorappe as discussed below.
Common Igneous Rocks Classified by SiO2 Content | |||||
---|---|---|---|---|---|
Type | Ultramafic < 45% SiO2 |
Mafic 45–52% SiO2 |
Intermediate 52–63% SiO2 |
Intermediate-felsic 63–69% SiO2 |
Felsic >69% SiO2 |
Volcanic rocks | Picrite basalt | Basalt | Andesite | Dacite | Rhyolite |
Subvolcanic rocks | Diabase (dolerite) | Microdiorite | Microgranodiorite | Microgranite | |
Plutonic rocks | Peridotite | Gabbro | Diorite | Granodiorite | Granite |
Cañón-Tapia (2010) suggests that the modelling of LIPs has been strongly influenced by the original definition of Coffin and Eldholm (1992; 1994), leading to “mythical thinking” in the assumption that large amounts of magma must have formed in a relatively short time; LIP eruptions were thus viewed in mainstream thinking as essentially melting events. However, Silver et al. (2006) had found that cratonic flood basalts in Southern Africa and Siberia could better be modelled as two-stage drainage events, involving (1) slow formation of reservoirs of molten basalt beneath the continental crust, followed by (2) rapid drainage through dikes created by abrupt changes in lithospheric stress. Cañón-Tapia’s (2010) model considers regions of partial melt to be a natural feature of the tectonic evolution of the earth, simply marking where mantle conditions lead to the melting of some of its constituent minerals. Cañón-Tapia (2010) suggests that such conditions apply generally below an average depth of 100 km beneath continents, coinciding with a globally detected seismic discontinuity, and can therefore occur in cratonic environments. He claims that such regions of partial melt (RPM) are responsible for volcanic activity around the world, whether or not related to a LIP. In sharp contrast with Coffin and Eldholm (1992; 1994) and Bryan and Ernst (2008), Cañón-Tapia concludes that extraordinary or abnormal mechanisms are not needed to explain the occurrence of LIPs, but does not see this as ruling out a mantle plume origin; instead, it suggests a wider scope in possible models of LIP origins than usually considered. This view, that LIPs represent the extreme of a range of intensity and magnitude of natural mechanisms underlying magmatism, is of some interest in the context of Flood modelling.
From a creation science perspective, however, the key practical questions regarding LIPs are: (1) the total amount of heat deposited in their formation; (2) eruption rates; (3) possible cooling modes, and associated cooling and crystallization timescales; (4) the relationship, if any, of LIPs with mass extinction episodes, in view of the proposal that LIPs have been a major cause of mass extinctions (for example, Hallam 2004; Wignall 2001; 2005), notably because of the massive flux of volatiles (CO2, SO2 etc.) into the environment expected from such large eruptions. Although important in the context of Flood-related versus conventional long-age interpretations of earth history, point (4) is beyond the scope of the present article and will not be discussed further here. We consider the first three questions in turn.
Heat deposited in the formation of LIPs
The total quantity of heat deposited in LIP formation can be estimated fairly straightforwardly given the total volume of rock involved; the biggest problem is in estimating the volume, especially for older LIPs, which as noted above may have been subject to erosion, burial, or tectonic fragmentation since emplacement (Bryan and Ferrari 2013; Ernst 2007). Typical densities of basaltic and silicic magmas are ~2,750 kgm–3 and 2,450 kgm–3 respectively (Bryan et al. 2010), but the large-scale rock density may be lower than the constituent solid rock densities because of high porosity, especially in the case of silicic LIPs, in which the dominant rock type is rhyolitic ignimbrite. This has a typical density range of 1,212–1,928 kgm−3, with porosity up to 50% (Moon 1993); for example, average values for tuff samples from the Alban Hills in Italy are ~1,540 kgm–3 for density and ~33% for porosity (Zhu et al. 2011).
An initial guide to the heat deposited in forming mafic LIPs with low porosity may be gleaned from the figures given by Furlong and Chapman (2013) for the heat deposited in the production of basaltic oceanic lithosphere, viz. 4 × 1014 Jm–2 in terms of area; given an assumed lithospheric thickness of 100 km, this equates to 4 × 109 Jm–3 in terms of volume. Furlong and Chapman (2013) do not cite sources for this figure. In contrast, LIP formation is essentially a crustal phenomenon. Consider the Cretaceous Ontong Java Plateau (or OJP)6 in the Western Pacific, the largest LIP on earth by volume. Its maximum thickness is 38 km, and its average thickness 32–33 km (Gladczenko, Coffin, and Eldholm 1997; Richardson, Okal, and Van der Lee 2000). The surface area of the OJP proper is approximately 2 × 106 km2 (Fitton et al. 2004); for the whole Ontong Java Nui complex, Ernst et al. (2021) cite an area of 6.41 × 106 km2. Bathymetry of the main OJP area is shown in fig. 7 and a sample of the vertical structure of the plateau at ODP Site 1185 is shown in fig. 8; it basically consists of alternating sections of massive basalts and pillow basalts overlain by sediment. Its profile in a typical transect shows a surface elevated only 2.5 km above the local ocean floor within a total vertical thickness of 35 km; it erupted under approximately 1 km of ocean water (Korenaga 2005; Roberge et al. 2005). Standard plume models and bolide impact models imply that it should have erupted subaerially and that it should have subsided much more than observed (Korenaga 2005; Roberge et al. 2005). Instead Korenaga (2005) proposed that the OJP formed by extensive melting of fertile eclogite (from recycled ocean crust) entrained by passively upwelling upper mantle material beneath or near a mid-ocean ridge; this model was criticized by Hoernle et al. (2010) on compositional grounds, who proposed their own thermochemical superplume model.
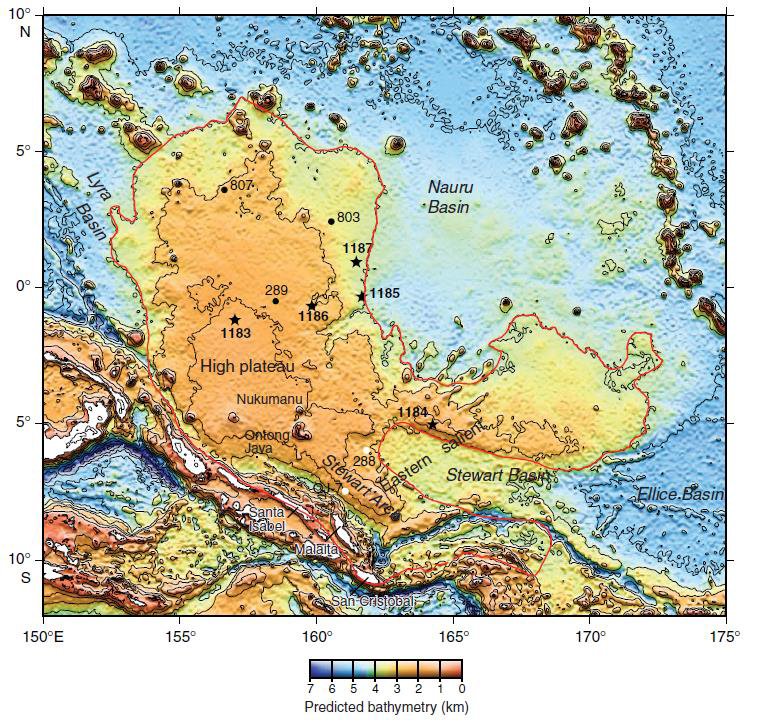
Fig. 7. Reproduction of fig. F1 in Mahoney et al. (2001). Predicted bathymetry (after Smith and Sandwell 1997) of the Ontong Java Plateau (outlined) showing the locations of sites drilled during Leg 192 (stars). Black dots = previous ODP and DSDP drill sites that reached basement. White dots = Site 288, which did not reach basement but bottomed in Aptian (Lower Cretaceous) limestone, and Site OJ-7, which was proposed for Leg 192 but not drilled. The bathymetric contour interval is 1,000 m.
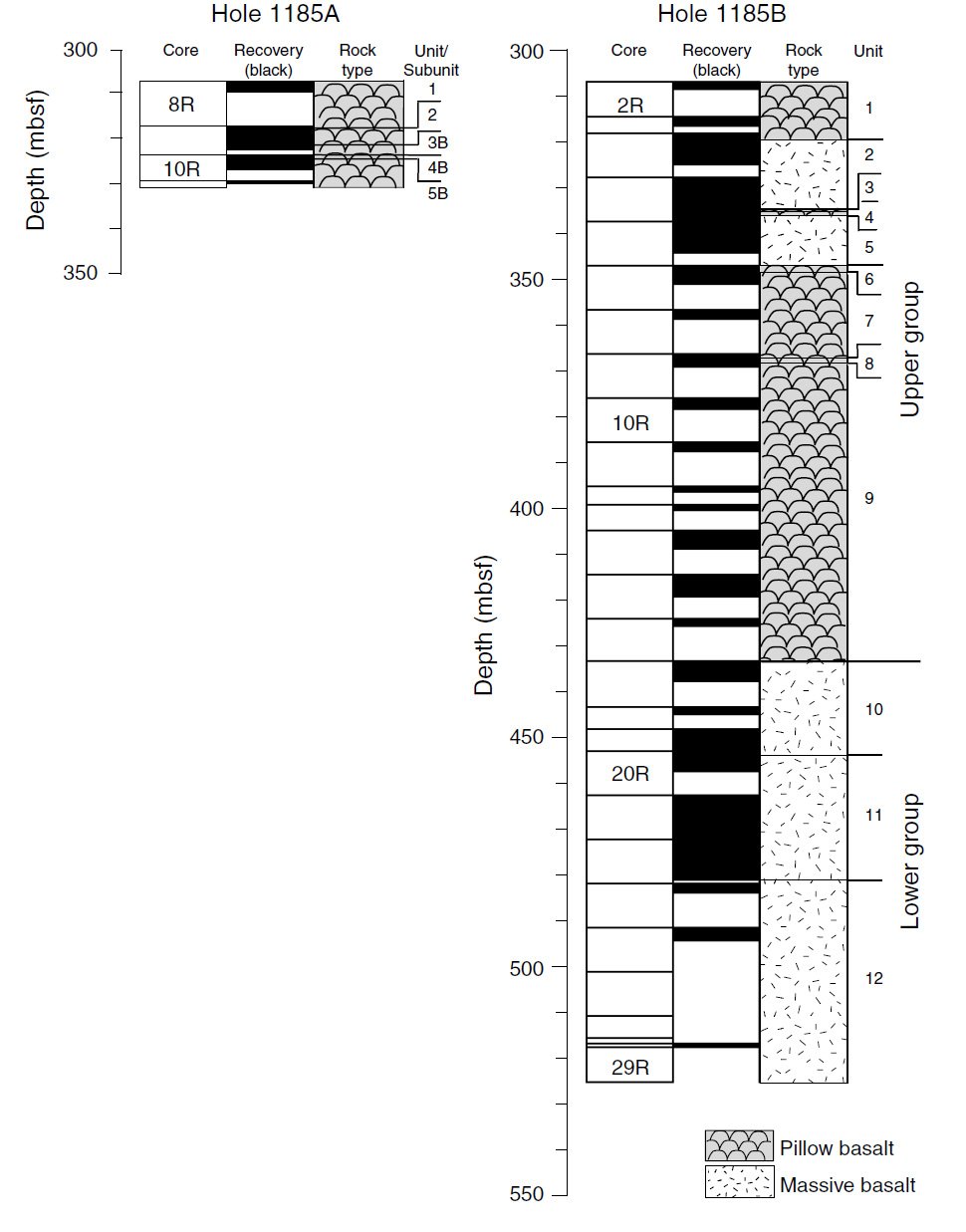
Fig. 8. Reproduction of fig. F26 in Mahoney et al. (2001). Lithological log of basement rock units at Ontong Java Site 1185; depth figures refer to meters below mean sea level. The upper and lower groups of units in Hole 1185B are distinct in their petrography, composition, state of alteration, and biostratigraphic age.
This question of subaerial versus subaqueous eruption, though not central here, is important in Flood geology because Genesis 7:19–20 implies that all mid-Flood volcanic activity would have been subaqueous. However, the Kerguelen Plateau/Broken Ridge LIP, in contrast to the OJP, is thought to have erupted subaerially (Frey et al. 2003), yet the major eruptions producing these formations occurred almost simultaneously, at a point corresponding to Aptian (early Cretaceous) rocks. This apparent conflict with Flood geology suggests the need for a wide-ranging investigation of the environmental conditions in which LIP eruptions occurred.
With the OJP in mind, consider the heat deposited by 1 kg of basaltic magma in the process of cooling from its eruption temperature, approximately 1,200°C (Lesher and Spera 2015), to its present-day average temperature assuming a near-surface melt fraction of 28% (Kerr and Mahoney 2007; Mahoney et al. 1993). We assume specific heats at constant pressure of 1,450 Jkg–1 K–1 for the liquid phase (Lesher and Spera 2015), and 1,050 Jkg–1 K–1 for the solid phase, a rough average for the range 100–1,000°C (Hartlieb et al. 2016), and latent heat of crystallization 4.2 × 105 Jkg–1 (Stacey and Davis 2008, table A.6). Assuming steady-state conditions the local Moho temperature may be estimated from the average geothermal heat flux through the OJP, 47 m Wm–2 (Richardson, Okal, and Van der Lee 2000), the thermal conductivity of basalt, 2.5 Wm–1 K–1 (Stacey and Davis 2008, table A.6) and an assumed crustal thickness of 33 km (see above). For an ocean floor temperature of 0°C, these figures give a credible Moho temperature of 620°C and hence an average temperature for the whole mass of plateau material of 310°C and an average heat release of 1.05 × 106 Jkg–1.
For an average basalt density of 2,900 kgm-3 (Stacey and Davis 2008, table A.6) this is equivalent to a total volumetric heat release of 3.05 × 109 Jm–3.
The estimated volume of the OJP, including the Manihiki and Hikurangi plateaus and the nearby Nauru and East Mariana Basins, is in the range 5.9–7.7 × 107 km3 (Kerr and Mahoney 2007). Neal, Coffin, and Sager (2019) give area and volume figures for three major oceanic LIPs, viz. Ontong Java (with Manihiki and Hikurangi), Shatsky Rise (NW Pacific, 1,500 km east of Japan) and Kerguelen Plateau/Broken Ridge (southern Indian Ocean/Southern Ocean). The total volume of these LIPs is in the range 5.9–9.0 × 107 km3. Taking the unit heat loss figure (3.05 × 109 Jm–3) derived above then gives a total heat deposition from the formation of these LIPs of between 1.8 × 1026 J and 2.7 × 1026 J. Ernst (2016) gives a total volume of oceanic LIPs, none lower than Triassic in the rock record, of ≈1.0 × 108 km3, which corresponds, assuming the same unit heat loss figure, to a total heat deposition of 3.0 × 1026 J. It is instructive to compare this figure, which represents the total heat deposited by oceanic LIPs during the Flood, with the thermal capacity of earth’s oceans. The total volume of ocean water today is 1.335 × 109 km3 (Eakins and Sharman 2010) and approximate average values for its density and constant-pressure specific heat capacity are 1,025 kgm-3 and 4,000 Jkg–1K–1 respectively (Nayar et al. 2016; Sharqawy, Lienhard, and Zubair 2010), implying a total thermal capacity of 5.5 × 1024 J/K. In the absence of any external heat loss mechanism the above heat deposition value would give a temperature rise averaged over the whole of earth’s oceans of 55°C.
Although continental flood basalt provinces (CFBs) are more accessible to geological investigation than oceanic LIPs, the heat deposited in their formation is difficult to estimate because the total emplaced volume is often highly uncertain. This may be because they are usually emplaced in and upon continental crust, which is typically thicker and more complex in structure than oceanic crust, and they may have been subjected to varying degrees of erosion. Furthermore petrological and geochemical studies are compromised by possible contamination of the magma by the continental crust and lithospheric mantle through which it passes (Fitton et al. 2004). Some CFBs are now in a very different configuration than when emplaced: for example, the Central Atlantic Magmatic Province (CAMP), the largest CFB on earth in terms of area, is represented by rocks in four continents, Africa, South America, North America, and Europe (Bertrand et al. 2014; Ernst et al. 2021), while the Paraná-Etendeka CFB is represented in both Africa and South America and in their Atlantic-facing continental margins (Bryan and Ferrari 2013; Gibson, Thompson and Day 2006; Peate 1997). The volumes cited for the largest Phanerozoic basaltic CFBs by Ernst (2016) are 4.5 × 106 km3 (Madagascar), 5.0 × 106 km3 (Karoo/Ferrar), and 4.0 × 106 km3 (Siberian Traps), together with an estimate of 2.0 × 106 km3 for the original CAMP emplacement (Marzoli et al. 1999). The sum total of these volumes is less than 2 × 107 km3. Even allowing for significant uncertainties in these volume estimates, and for the possible existence of as yet unidentified Phanerozoic CFBs, the total volume is unlikely to exceed 3 × 107 km3, representing no more than a 30% addition to the heat deposited by oceanic LIPs. Since heat released in the sub-aerial emplacement of magma will first be transferred to the atmosphere and from there to the oceans and (by radiation) to space, only a proportion of it will contribute to heating the oceans. In comparison with basaltic LIPs, silicic LIPs will contribute very little in terms of total heat deposition because of their much smaller total volume. Hence the gross heat deposition due to LIPs emplaced during the Flood can hardly exceed 4.0 × 1026 J. If all of this heat due to LIP emplacement were to be dissipated by purely natural mechanisms within the one year of the Flood, the necessary rate of heat loss would be 72 times the gross solar input to the earth (see table 1). Even if spread over hundreds of post-Flood years, this additional heat flow would drastically affect earth’s climatic conditions.
When considered in isolation, the above estimates (which exclude the heat released globally in ocean floor cooling) give an idea of the potentially dramatic impact of heat deposited by LIPs which erupted and were emplaced during and after the Flood. However the total amount of heat involved (not exceeding 4.0 × 1026 J) is insignificant in comparison with the heat deposition involved in forming the ocean floors (1.4 × 1029 J). Thus the heat released in the formation of LIPs adds relatively little to the overall problem of discerning the mechanism whereby the ocean floors must have cooled during and after the Flood. However, the local impact of the heat from LIPs, which in many cases must have been released within a matter of days or weeks at most, would have been highly dramatic in the absence of some special, hitherto unrecognized, cooling mechanism.
Eruption rates
Magma eruption rates and cooling timescales associated with LIPs are of interest in Flood modelling. Bryan et al. (2010) review the magnitudes and eruption rates of the individual eruptions responsible for the formation of LIPs; see also White et al. (2009). Volumetrically, these eruptions, both basaltic and silicic, are the largest in earth history. Their emplaced volumes can exceed 1,000 km3 in terms of dense rock equivalent, and their magnitudes can exceed M8 (see Mason, Pyle, and Oppenheimer 2004 for an explanation of the logarithmically-based M index), which corresponds to a total mass of 1015 kg, leading to basaltic lava flow fields and silicic ignimbrites covering areas of 104–105 km2. Although flood basaltic and silicic eruptions are comparable in magnitude, they erupt and undergo emplacement quite differently. Flood basaltic eruptions are mainly effusive and Hawaiian-Strombolian7 in style, with magma flow rates of ~106–108 kg s−1 and durations estimated at years to decades, usually resulting in compound pāhoehoe lava flow fields; typically pāhoehoe lava flows are emplaced by inflation, the injection of molten lava beneath a solidified crust which provides a significant degree of thermal insulation (Self, Keszthelyi, and Thordarson 1998). For example, the Roza member of the Columbia River Flood Basalt Group was estimated by Thordarson and Self (1998) to have resulted from an eruption which produced several inflated sheet lobes (see fig. 9) and lasted in total ~14 years, implying an average eruption rate of 4.2 × 106 kg s–1. Pāhoehoe has a continuous smooth, billowy, or ropy crust, while ‘a‘ā, which often develops downstream from a pāhoehoe flow, has a brecciated rough, spiny, or clinker-like surface. In terms of both areal coverage and total volume, pāhoehoe flows dominate basaltic lavas in both subaerial and submarine environments on earth. Such flows can be extremely long, for example, the Rajahmundry Trap lavas (associated with the Deccan Basalt Group) near the east coast of India, which indicate that lava flowed for about 1,000 km from its source (Self et al. 2008).
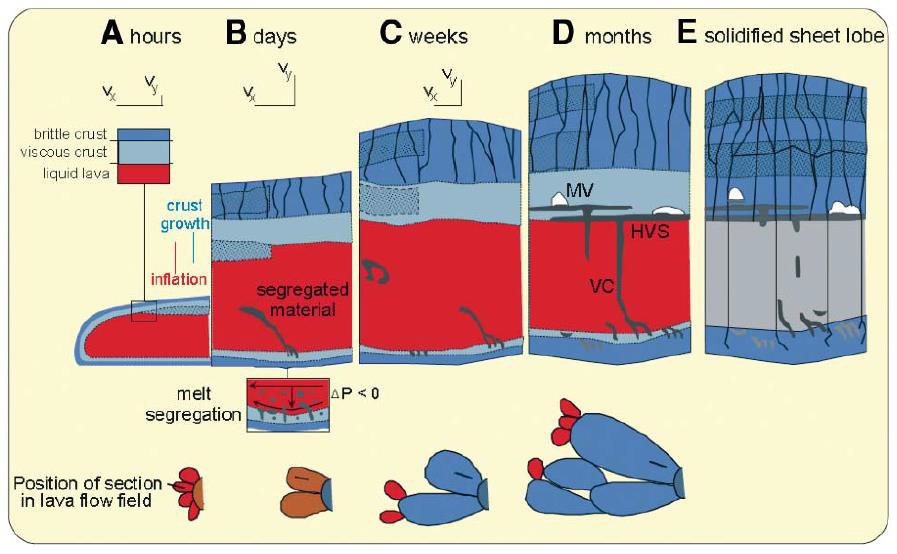
Fig. 9. Reproduction of fig. 4 in Self, Schmidt, and Mather (2014) showing the development of a pāhoehoe basalt lava flow field over time as the lava sheet lobes grow outward and downslope by advance. Lobes also coalesce and thicken by inflation. Vx and Vy show proportions of lateral and vertical expansion of flow lobe. Inset shows localized separation of flow lines leading to drops in internal pressure (P) and vesiculation at the basal crust-core boundary. Schematic plan view of growing lava flow field is given beneath each stage. MV—megavesicles; HVS—horizontal vesicular sheets; VC—vesicle cylinders. This scheme applies to flood basalt lava flow fields (after Thordarson and Self 1998).
Submarine lavas, the most abundant volcanic products on earth, are closely related to pāhoehoe in their style of emplacement, but may have a variety of surface morphologies. Inflation can convert lava flows only 20–30 cm thick to sheets of 1–5 m thickness (Hon et al. 1994; Self, Keszthelyi, and Thordarson 1998). In a detailed study of the Deccan and Columbia River Provinces, Self, Mittal, and Jay (2021) found a modal lobe thickness of 8 m in Deccan formations with abundant thin lava-lobes, while the mode for formations dominated by sheet lobes was only 17 m; sheet-lobes up to 75–80 m are rare in the Deccan and Columbia River Provinces, and thicknesses >100 m are exceptional globally. Although pāhoehoe lava flows move more slowly and are visually less striking than ‘a‘ā flows, they can continue for long periods of time and produce very large basalt flow fields (Self, Keszthelyi, and Thordarson 1998). Conversely, most silicic eruptions are moderately to highly explosive, taking the form of pyroclastic fountains (occasionally Plinian) with discharge rates of 109–1011 kg s−1 which produce ignimbrites. Durations for the large-magnitude silicic eruptions are currently unknown: at discharge rates of 109 kg s−1, equivalent to the peak of the 1991 Mt Pinatubo eruption, the largest would take many months to discharge >5,000 km3 of magma. The generally simple deposit structure suggests short (hours to days), high intensity (~1011 kg s−1) eruptions, probably with interruptions in some cases.
For a present-day comparison, note that the Yellowstone magmatic system, which is silicic in character, consists of two magma chambers: the upper-crustal magma body has a volume of 1.0 × 104 km3 with estimated melt fraction ~9%, and the lower-crustal magma body a volume of 4.6 × 104 km3 with melt fraction ~2% (Huang et al. 2015). Mittal, Richards, and Fendley (2021) and Mittal and Richards (2021) have investigated in detail the magmatic architecture of CFBs, with specific focus on the Deccan Traps, and conclude that the dynamics of these eruptions are inconsistent with just a few large magma reservoirs and instead require a network of smaller magma bodies feeding each eruption.
Modes and timescales of LIP cooling
The LIP eruption rates cited in the preceding section are all taken from the mainstream literature, the underlying assumption being that earth history spans billions of years. Working within a biblical framework, we naturally ask whether the long-age paradigm has influenced these figures. In general, it seems that they are based on natural constraints on eruption rates (for example, magma conduit dimensions, magma composition, temperature, pressure and density, effective magma viscosity, magma chamber volume etc.) and on observations of present-day volcanic activity and magmatic systems. However, since all LIPs are considered to have been emplaced within a time-envelope measured in millions of years, it is plausible, at least for effusive basaltic eruptions, that the quoted eruption rates are unrealistically low. Furthermore, the inference of pulsed eruptions in Bryan and Ernst’s (2008) definition of LIPs, which implies interspersed periods of quiescence of undetermined duration, is probably based on the necessity of stretching the total emplacement time for particular LIPs to suit the conventional geological timescale. Snelling and Woodmorappe (2009) cite an example of such timescale stretching in the case of the Cordillera Blanca batholith of north-west Peru investigated by Petford, Kerr, and Lister (1993), who estimate a batholith filling time of 350 years but suggest that active filling must have been divided into small events interspersed by periods of recharge. Since Petford, Kerr, and Lister (1993) cite mineral cooling ages, based on K-Ar and Ar-Ar analyses, of between ~6 and 3.0 Ma for the central region of the intrusion, this implicitly requires periods of recharge lasting typically ~104–105 years each. This is quite out of proportion with the active filling events. It seems likely that this timescale stretching in deference to isotopic age determinations is the norm in the LIP literature. Further investigation of this aspect by creation scientists may well prove worthwhile.
This analysis highlights vital questions from a creation science perspective, viz. how, and how rapidly, LIPs have formed and cooled to their present state. The same questions with regard to granite and granitic plutons were addressed by Snelling and Woodmorappe (1998; 2009) and Snelling (2008), who demonstrated how these formations could well have been produced within biblically-compatible timescales. Snelling and Woodmorappe (1998; 2009) also consider entablatures and colonnades, classic features of flood basalt provinces. Entablatures typically cool at 1–10°C per hour (Long and Wood 1986), and colonnades ten times more slowly, but shorter cooling times for both are also compatible with the data provided that the relative cooling rates of these features differ by at least an order of magnitude (DeGraff, Long, and Aydin 1989). However, the work of Snelling and Woodmorappe (1998; 2009) and Snelling (2008) does not address the question of the total amount of heat deposited: their implicit assumption is that the heat capacity of the global environment is so large that its temperature is not significantly raised by the emplacement and cooling of granitic formations. The constraint of a biblically-compatible timescale makes this an especially pertinent issue in Flood geology because this heat must be removed very quickly from the surface rocks and biosphere, just as in the case of the much bigger problem of ocean floor cooling discussed above.
Uniformitarian geologists now recognize that granitic plutons and batholiths (the largest plutons) must have been produced in geologically short times, 105 years or less in conventional terms, sometimes considerably less (Petford et al. 2000). The most popular model of granite formation before the 1990s, viz. as diapirs, large globular masses of magma ascending through the crust by virtue of being lighter (less dense) than the surrounding rocks, has been discredited: transport of granitoid magmas in this manner is ruled out on thermal and mechanical grounds, and field evidence for it is lacking (for example, Biggs and Annen 2019; Clemens 2005; Clemens and Mawer 1992; Petford et al. 2000; Petford, Kerr, and Lister 1993). Rather, granitic magma supply to forming plutons takes place largely through dikes, mainly vertical fractures which can readily propagate upwards under the thermal and mechanical stresses induced by the rising magma, a much faster process than envisaged for the alleged rise of diapirs. The essential steps in continental granite magmatism are partial melting, segregation of melt from solid, ascent, and emplacement (Petford et al. 2000); the rate-limiting step is partial melting in the lower crust, which is now understood to take only years to decades (Snelling 2008). This could have taken place in the pre-Flood period, especially if accelerated decay of crustal radionuclides was occurring. Water is a major factor: crystallization within the magma increases the water content of the residual melt, which reduces its viscosity. Furthermore, meteoric water percolates into a developing pluton via the cooling/cracking front propagating inwards from its outer surface, which helps to maintain its permeability and induce vapor pressures leading to fracturing of the country rock. This in turn facilitates hydrothermal convection which rapidly dissipates heat released by the cooling, solidifying magma (Snelling and Woodmorappe 1998). Tectonic squeezing and pumping, which would have been especially vigorous during the Flood, would have aided the processes of segregation, ascent, and emplacement, which could thus have been completed within days (Snelling 2008; Snelling and Woodmorappe 2009).
As suggested above, the question arising from this understanding of granitic pluton formation and the related timescales is whether it can be applied to LIPs generally. Granitic plutons are intrusive, whereas LIPs are classified by Eldholm and Coffin (2000) into four basic types, viz. (1) continental flood basalts, (2) oceanic plateaus, (3) ocean basin flood basalts and (4) volcanic passive continental margins. The basic structure of each type is shown in fig. 10, and a structural comparison of two continental flood basalt provinces with two silicic LIPs in fig. 11. In general LIPs include both intrusive and extrusive components, the latter being emplaced on top of existing crust and fed by systems of dikes and sills. Magma production beneath the crust and its rise through the crust are therefore essentially similar processes to those involved in forming granitic plutons and batholiths, although LIPs are formed in a greater variety of environments and are subject to a wide range of the controlling parameters, including magma temperature, composition and viscosity, water content, conduit dimensions, crustal rock thermomechanical properties, and so on. Magma reservoirs and their wall rocks span a vast array of rheological properties, covering as much as 25 orders of magnitude from high viscosity, sub-solidus crustal rocks to magmatic fluids (Sparks et al. 2019). In the case of continental rifting, the rate and form of rifting are also important parameters. The most obvious differences lie in the above-surface region, that is, depending on whether emplacement occurs subaerially or subaqueously. In a subaerial environment, cooling will occur via radiation, convection, and the action of meteoric water, whereas underwater, there will be cooling via convection and hydrothermal activity; heat loss rates are considerably higher underwater than in a subaerial setting (for example, Deschamps et al. 2014).
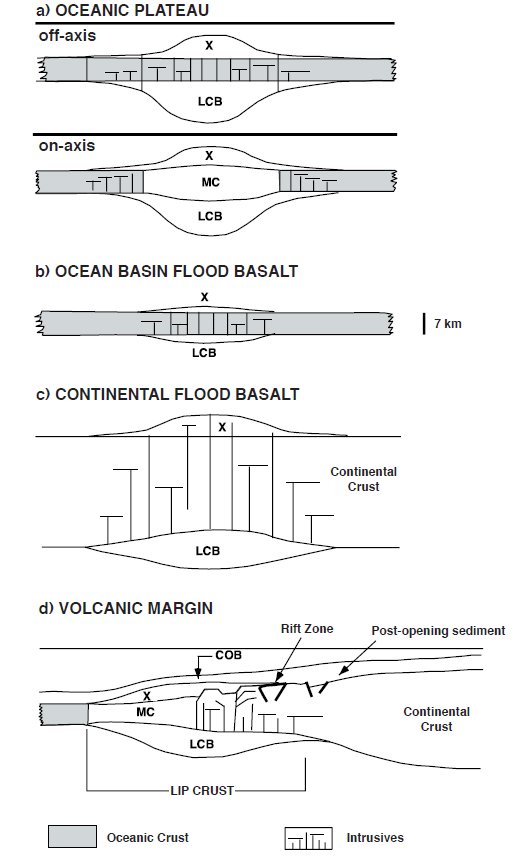
Fig. 10. Reproduction of fig. 2 in Coffin et al. (2006). Schematic of LIP plate tectonic settings and gross crustal structure. LIPs are emplaced in a variety of plate tectonic settings, yet are characterized by a common three-layer crustal structure, although crustal thickness varies considerably. LIP crustal components are: extrusive upper crust (X), middle crust (MC), and lower crustal body (LCB). Normal oceanic crust, 7 km thick, is grey. Horizontal scale varies from a few hundred to more than a thousand kilometers. The acronym COB is not defined by Coffin et al. (2006), but must represent covering material which emplaced after the relevant volcanism, possibly sediment cover.
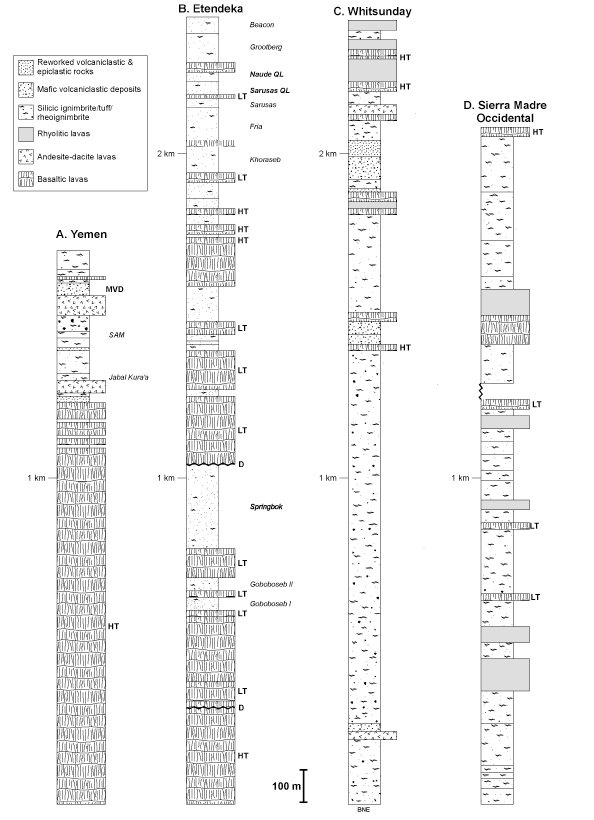
Fig. 11. Reproduction of fig. 5 in Bryan (2007). Generalized composite stratigraphic sections comparing two continental flood basalt provinces (Afro-Arabian and Etendeka) with two Silicic LIPs (Whitsunday and Sierra Madre Occidental). Dykes, sills, and other intrusions are not shown.
Modes and timescales of cooling within LIPs will, in general, be strongly affected by their layered structure, which results from the pulsed nature of the volcanism. The durations of the eruptive and quiescent intervals, as well as the quantity and thermophysical properties of the erupting magma and its emplacement environment (which may differ between eruptions), will all have an effect. Order-of-magnitude estimates of the timescale for cooling a layer of low-porosity basalt by heat conduction alone give figures of ~12 days for a thickness of 1 m, ~3.2 years for a thickness of 10 m, and so on, but these figures are significantly reduced if water can penetrate via fractures. In particular, in oceanic settings where the magma cools and solidifies underwater, ocean water is forced under pressure into downward-penetrating fractures; at 1 km depth, for example, the pressure is of the order of 100 bar. This pressurized water, in combination with water from the rising magma, sets up cooling hydrothermal flows which transfer heat from the magma to the oceans, just as happens at ocean spreading centers. In addition to the details of layering within LIPs (layer thicknesses, compositional variations, contact surface topology, and petrography, etc.), a further aspect obviously worth investigating is the pattern and scale (spacing and width) of fractures within LIP rock formations: the distance between fractures is important in assessing a cooling timescale based on heat diffusion, while fracture width is important in constraining the flow of cooling hydrothermal waters. All this is a matter for future research; the considerations regarding cooling modes and timescales for oceanic crust discussed in the previous section may not fully apply to oceanic LIPs, even less for continental flood basalt provinces.
Such research will require investigation of the key cooling processes and associated timescales at all relevant scales (from crustal down to submillimeter scales) in different types of LIP as compared with granitic intrusions, including:
(a) mafic continental flood basalt provinces (for example, the CRBG, the Deccan Traps and the Siberian Traps);
(b) silicic LIPs (for example. the Sierra Madre Occidental, Chon Aike, and Whitsunday Provinces);
(c) Oceanic plateaus and flood basins (for example, the Ontong Java Nui, Kerguelen/Broken Ridge Provinces etc).
In some cases, notably in continental flood basalt provinces, field observations of layer thicknesses, texture and chemistry, together with conduit dimensions (length, diameter, spacing etc.), it has been possible to constrain emplacement and cooling timescales. For example, Reidel (1998) concludes that the main part of the CRBG was emplaced within days to weeks, while the peripheral regions took months to years; in a later publication the same author (Reidel 2015) cites typical times of between a few days and a few years for emplacement of inflated sheet flows within the CRBG. Oard (1999), referring to the work of Ho and Cashman (1997) on the cooling rate of the CRBG Ginkgo flow, argues for very rapid emplacement of the flows which produced the CRBG, possibly of order one day for each flow; this in turn allows for emplacement of all 300 flows making up this Group within about one year. Woodmorappe and Oard (2002) undertook field studies of the CRBG, from which they claimed evidence of rapid underwater extrusion, rapid cooling, and rapid succession of lava flows and suggested that such studies should be extended to other relatively accessible CFBs.
Although methods based on radioactive decay are important in this context, and often critical in determining interpretations such as the duration of LIP volcanism (for example, Gibson, Thompson, and Day 2006; Schmitt 2011; Thiede and Vasconcelos 2010), a wide variety of other methods are used, from observations of present-day volcanic activity to modelling of lava sheet flows (including inflation), modelling of flow and heat transfer in 2D conduits (corresponding to dikes and sills), calculation of heat and chemical diffusion (implying the use of diffusion chronometry) over a range of scales, and the use of chemical/petrological markers to ascertain temperatures and timescales of crystallization (Cooper 2019; Costa, Shea, and Ubide 2020). Costa, Shea, and Ubide (2020) note in the case of silicic calderas that crystallization-based timescales can be almost two orders of magnitude shorter than the timescales deduced from uranium-thorium isotope disequilibria in zircon. Bindeman et al. (2020) analyzed a δ18O dataset for dikes and lavas to investigate the thermal effects of hydrothermal flows in the CRBG: higher temperatures are indicated by more negative δ18O values. Their finding that dikes on average are 1–2‰ more negative in δ18O than basalt flows was interpreted as due to heated meteoric waters cooling within the crust. The largest depletion was found around and inside a 10 m-thick feeder dike which likely served as a magma conduit for 4–7 years, based on the extent of heating and melting of its host rocks. A hydrothermal system produced by this dike extended up to 100 m into the surrounding bedrock; Bindeman et al. (2020) infer that this operated for ~150 years after magma flow had ceased. Contact-hydrothermal δ18O depletion of rocks around dikes in the sampled region occupies 500–600 km3, which scaled to the CRBG footprint constitutes 31,000 km3 of low-δ18O rocks; the latter figure is approximately 15% of the total CRBG emplaced volume.
At the largest scales the building blocks of granitic plutons are extensive (103–105 km2), sheet-like lava flow fields (Petford et al. 2000); their lengths and thicknesses follow scale-independent power-law relationships (McCaffrey and Petford 1997), who argue that the physical meaning behind this relationship is best explained if both laccoliths (blister-shaped masses of igneous rock intruded between sedimentary layers) and sheeted plutons are a result of similar ascent and emplacement mechanisms. According to Bryan et al. (2010), pāhoehoe is the predominant style of lava flow field forming the flood basalts in LIPs. Each flow field is interpreted as the product of one sustained eruptive event producing several major lava flows consisting of multiple sheet-like flow lobes—hence broadly similar to granitic plutons. The major sheet lobes contain most of the lava volume, and in the CRBG are typically 20–30 m thick, and several kilometers wide. In the Karoo and Etendeka flood basalt Provinces, however, investigations have revealed only a few lava flows >20 m thick, suggesting that the majority of the flood lava volume is not always expressed in thick lava flow units. The largest LIPs contain sheets of more than 106 km2, for example, the Ontong Java-Hikurangi-Manihiki plateaus, which have a total pre-rift areal extent of ~3.5 × 106 km2, and a maximum crustal thickness of 30 km (Bryan et al. 2010).
Submarine basaltic lava flows, which account for more than 60% of volcanic rocks on the earth’s crust, are less accessible to observation. Although analogue laboratory experiments and recent observations have revealed a variety of morphologies related to eruption rates (Fundis et al. 2010; Gregg and Fink 1995; Rubin et al. 2012), there is relatively little data available on the internal structure of basaltic oceanic LIPs down to the same resolution as is available for continental flood basalts. In contrast to CFBs the large-scale stratigraphy of silicic eruptive units generally appears to be simpler, as exemplified by the Paraná–Etendeka quartz latites which outcrop as relatively featureless extensive sheets with simple internal structure and jointing, leading to the interpretation as single flow or cooling units (Bryan et al. 2010; Milner, Duncan, and Ewart 1992).
Conclusions and Recommendations for Future Work
The main conclusion of this article is that the total amount of geological heat deposited in the formation of the ocean floors and of LIPs is overwhelming: it cannot be removed from the biosphere within a biblically-compatible timescale by known natural processes. Using CPT-style Flood models as our theoretical framework, no more than a tiny fraction of the total could have been released into the atmosphere and oceans during and after the Flood. Given that the highest bulk ocean temperature in the early Cenozoic did not exceed 13°C in contrast with the present-day value of ~2°C (Worraker 2018; the lower figure of 2°C may be taken as a representative pre-Flood minimum temperature), the total heat absorbed by the oceans, earth’s main environmental heat sink, would have been of order 6 × 1025 J at most, assuming a thermal capacity of 5.5 × 1024 J/K (as estimated above). This is only 0.04% of the total heat deposition: the remaining 99.96% must have been removed or absorbed elsewhere. It seems that this must have been accomplished by some special, hitherto unrecognized mechanism.
However, it is important to appreciate that our inability to identify an acknowledged mechanism for removing the excess heat deposited during and after the Flood, an issue first identified over 35 years ago (Baumgardner 1986), is only a problem in the sense that it represents the limited nature of our human understanding. In a biblical context there is no fundamental problem because God purposely brought about the Flood (Genesis 6:17) as a judgment on the wicked human race of Noah’s day and covenanted with Noah to preserve human and animal life through the cataclysm (Genesis 6:18). He sovereignly accomplished both objectives, implying that environmental temperatures could not have risen beyond biological endurance limits. The only real problem is our current lack of understanding of how this was accomplished; the Flood account in Genesis 6–9 does not tell us directly whether supernatural processes were involved, though it seems very likely that they were. The same basic issue arises in connection with the topics to be covered in Parts 5 (heat due to Accelerated Nuclear Decay) and 6 (heat due to bombardments from space) of this series, and will be considered at greater length in Part 7.
A survey of the literature on the thermal history of earth’s mantle in terms of potential temperature has shown that there has been a general cooling of approximately 150°C since the lower Palaeoproterozoic, that is, through and since the Flood, and that the range of mantle potential temperatures at any one stratigraphic level has been ~170°C throughout. There is no obvious marker in the geological record of major global heating or cooling events corresponding to critical points within a CPT-style Flood scenario. These conclusions are based on the usual uniformitarian interpretation of stratigraphy as defining a sequence in time, though not necessarily the conventionally-accepted absolute timescale. A systematic reanalysis of the provenance of the rock samples used in the key publications could, in principle, be undertaken from the perspective of Flood geology to check these conclusions, but this would be enormously laborious and seems unlikely to produce useful results.
Although no clear solution to the so-called heat problem associated with Flood and post-Flood magmatism has been identified in this article, a number of related geological issues have been noted. Consequently a number of potentially worthwhile lines of future enquiry from a creation science perspective have been identified, which we enumerate below; note that these do not all relate directly to the problem of Flood heat and its removal, but they are of concern in the broader context of Flood geology.
- The model of ocean floor cooling used by Worraker and Ward (2018) could be revisited using a wide range of input parameters to check how closely its output could be made to match with observation, viz. bathymetry, or ocean floor topography, and ocean floor heat flux data. The results of such an exercise may indicate how far a model of this kind would need to be modified to predict anything approaching reality, or if indeed that is possible.
- Diffusion chronometry is an important technique for estimating timescales associated with the formation and chemical history of igneous rocks but has attracted relatively little interest from creation scientists except in certain special cases, notably the diffusion of helium in zircons (Humphreys 2005). A systematic review of the literature would seem worthwhile, especially since in several cases timescales ascertained by diffusion chronometry are in conflict with—specifically, shorter than—timescales deduced from disequilibria in radioactive decay series.
- Glazner (2021) has highlighted the difficulty in conventional geology in modelling the structure and dynamics of magma chambers because the predicted rapid crustal thickening and surface uplift are not observed. This suggests that an investigation of the available data relating to this problem from a creation science perspective may prove fruitful.
- The origin and nature of the two LLSVPs (Tuzo and Jason) on the core-mantle boundary is still a contentious question, to which a definitive answer cannot at present be given. However the recent CPT-based modelling work of Navarro (2021) represents a positive step towards a creation science understanding of these enormous mantle structures. Future developments in seismic tomography, especially in terms of improved resolution deep in the mantle, and further geochemical and mineral physics investigations relevant to this question, should be followed closely with a view to establishing a more complete description compatible with biblical constraints.
- Because of the pulsed nature of LIP eruptions and the generally undetermined duration of quiescent intervals between eruptions, an investigation of the relevant field data (geological, geophysical, and geochemical) for a number of LIPs would seem worthwhile. In cases already noted there is a suspicion that the duration of quiescent intervals is inflated because of the underlying belief in time spans measured in millions of years for the eruption and emplacement of LIPs.
- LIP eruptions have often been proposed in the geological literature as a major cause of mass extinctions. It is not clear how the implied cause-effect relationship might operate during the Flood. This suggests the need for a study of the evidence supporting the inference of a mass extinction, its character and extent, and its temporal relationship with an LIP eruption (if any) in individual cases.
- Flood geology naturally predicts that mid-Flood volcanic eruptions must have occurred underwater. Since this prediction apparently conflicts with observation in the case of certain LIPs (for example, the Kerguelen Plateau/Broken Ridge system), an investigation of the eruption environment of Phanerozoic LIPs, notably Mesozoic systems, is recommended with a view to resolving cases of apparent conflict and establishing the broad picture of LIP eruption environments during and after the Flood.
Acknowledgements
Paul Garner provided many of the references cited in this article. Richard Ward and Russell Humphreys assisted with the calculation of the generalized Stefan-Boltzmann coefficient in 4D hyperspace (Appendix 3). General advice regarding volcanism and plutonism, notably the cooling of large bodies of igneous rock, was provided by Birgir Óskarsson and Ben Clausen. The author acknowledges with thanks the assistance of these scientists.
References
Aarons, Sarah M., Jesse R. Reimink, Nicolas D. Greber, Andy W. Heard, Zhe Zhang, and Nicolas Dauphas. 2020. “Titanium Isotopes Constrain a Magmatic Transition at the Hadean-Archean Boundary in the Acasta Gneiss Complex.” Science Advances 6, no. 50 (9 December): eabc9959. doi: 10.1126/sciadv.abc9959.
Abbott, Dallas, Lee Burgess, John Longhi, and Walter H. F. Smith. 1994. “An Empirical Thermal History of the Earth’s Upper Mantle.” Journal of Geophysical Research 99, no. B7 (10 July): 13835–13850.
Alnes, Haavard, Finn Ravndal, and Ingunn Kathrine Wehus. 2007. “Black-Body Radiation with Extra Dimensions.” Journal of Physics A: Mathematical and Theoretical 40, no. 47 (23 November): 14309–14316.
Anderson, Don L., and James H. Natland. 2005. “A Brief History of the Plume Hypothesis and Its Competitors: Concept and Controversy.” In Plates, Plumes and Paradigms, edited by Gillian R. Foulger, James H. Natland, Dean C. Presnall, and Don L. Anderson. Geological Society of America Special Paper 388: 119–145.
Arndt, N., C. Ginibre, C. Chauvel, F. Albarède, M. Cheadle, C. Herzberg, G. Jenner, and Y. Lahaye. 1998. “Were Komatiites Wet?” Geology 26, no. 8 (August 1): 739–742.
Asimow, Paul D. 2017. “A Measure of Mantle Melting.” Science 355, no. 6328 (3 March): 908–909.
Austin, Steven A., John R. Baumgardner, Andrew A. Snelling, Larry Vardiman and Kurt P. Wise. 1994. “Catastrophic Plate Tectonics: A Global Flood Model of Earth History.” In Proceedings of the Third International Conference on Creationism, edited by R. E. Walsh, 609–621. Pittsburgh, Pennsylvania: Creation Science Fellowship.
Avants, Megan, Thorne Lay, and Edward J. Garnero. 2006. “A New Probe of ULVZ S-wave Velocity Structure: Array Stacking of ScS Waveforms.” Geophysical Research Letters 33, no. 7 (April): L07314. doi: 10.1029/2005GL024989.
Ball, Will T., Yvonne C. Unruh, Natalie A. Krivova, Sami Solanki, and Jerald W. Harder. 2011. “Solar Irradiance Variability: A Six-Year Comparison Between SORCE Observations and the SATIRE Model.” Astronomy and Astrophysics 530, A71 (June). doi: 10.1051/0004-6361/201016189.
Barrick, William D. 2008. “Noah’s Flood and Its Geological Implications.” In Coming to Grips with Genesis: Biblical Authority and the Age of the Earth, edited by Terry Mortenson, and Thane H. Ury, 251–281. Green Forest, Arkansas: Master Books.
Barrow, John D., and Frank J. Tipler. 1986. The Anthropic Cosmological Principle. Oxford, United Kingdom: Oxford University Press.
Baumgardner, John R. 1986. “Numerical Simulation of the Large-Scale Tectonic Changes Accompanying the Flood.” In Proceedings of the First International Conference on Creationism, edited by R. E. Walsh, C. L. Brooks, and R. S. Crowell, 17–28. Pittsburgh, Pennsylvania: Creation Science Fellowship.
Baumgardner, John R. 2000. “Distribution of Radioisotopes in the Earth.” In Radioisotopes and the Age of the Earth: A Young-Earth Creationist Research Initiative, edited by Larry Vardiman, Andrew A. Snelling, and Eugene F. Chaffin, 49–94. El Cajon, California: Institute for Creation Research, and Chino Valley, Arizona: Creation Research Society.
Baumgardner, John R. 2003. “Catastrophic Plate Tectonics: The Physics Behind the Genesis Flood.” In Proceedings of the Fifth International Conference on Creationism, 113–126. Pittsburgh, Pennsylvania: Creation Science Fellowship.
Baumgardner, John. 2013. “Global Tectonics—Clarity, Not Confusion.” Journal of Creation 27, no. 1 (April): 99–106.
Bédard, Jean H. 2018. “Stagnant Lids and Mantle Overturns: Implications for Archaean Tectonics, Magmagenesis, Crustal Growth, Mantle Evolution, and the Start of Plate Tectonics.” Geoscience Frontiers 9, no. 1 (January): 19–49.
Bercovici, David. 2003. “The Generation of Plate Tectonics From Mantle Convection.” Earth and Planetary Science Letters 205, no. 3–4 (10 January): 107–121.
Bercovici, David, Yanick Ricard, and Mark A. Richards. 2000. “The Relation Between Mantle Dynamics and Plate Tectonics: A Primer.” In The History and Dynamics of Global Plate Motions, edited by M. Richards, R. Gordon, and R. van der Hilst, American Geophysical Union Geophysical Monograph 121: 5–46.
Bertrand, Hervé, Michel Fornari, Andrea Marzoli, Raúl García-Duarte, and Thierry Sempere. 2014. “The Central Atlantic Magmatic Province Extends into Bolivia.” Lithos 188: 1 February: 33–43.
Biggs, Juliet, and Catherine Annen. 2019. “The Lateral Growth and Coalesence of Magma Systems.” Philosophical Transactions of the Royal Society A 377, no. 2139 (25 February): 20180005. doi: 10.1098/rsta.2018.0005.
Bindeman, I. N., N. D. Greber, O. E. Melnik, A. S. Artyomova, I. S. Utkin, L. Karlstrom, and D. P. Colón. 2020. “Pervasive Hydrothermal Events Associated With Large Igneous Provinces Documented by the Columbia River Basaltic Province.” Scientific Reports 10 (June): 10206. doi: 10.1038/s41598-020-67226-9.
Bono, Richard K., John A. Tarduno, and Hans-Peter Bunge. 2019. “Hotspot Motion Caused the Hawaiian-Emperor Bend and LLSVPs Are Not Fixed.” Nature Communications 10 (29 July): 3370. doi: 10.1038/s41467-019-11314-6.
Bown, Jonathan W., and Robert S. White. 1994. “Variation With Spreading Rate of Oceanic Crustal Thickness and Geochemistry.” Earth and Planetary Science Letters 121, nos. 3–4 (February): 435–449.
Britannica. 2021. “Six Types of Eruptions.” https://www.britannica.com/science/volcano/Six-types-of-eruptions.
Brown, W. 2020. In the Beginning: Compelling Evidence for Creation and the Flood, Center for Creation Science. https://www.creationscience.com/onlinebook/HydroplateOverview.html.
Bryan, Scott E. 2007. “Silicic Large Igneous Provinces.” Episodes 30, no. 1 (March): 20–31.
Bryan, Scott E., and Richard E. Ernst. 2008. “Revised Definition of Large Igneous Provinces (LIPs).” Earth-Science Reviews 86, nos. 1–4 (January): 175–202.
Bryan, Scott E., Ingrid Ukstins Peate, David W. Peate, Stephen Self, Dougal A. Jerram, Michael R. Mawby, J. S. (Goonie) Marsh, and Jodie A. Miller. 2010. “The Largest Volcanic Eruptions on Earth.” Earth-Science Reviews 102, nos. 3–4 (October): 207–229.
Bryan, Scott E., and Luca Ferrari. 2013. “Large Igneous Provinces and Silicic Large Igneous Provinces: Progress in our Understanding Over the Last 25 Years.” GSA Bulletin 125, nos. 7–8 (January 1): 1053–1078.
Burke, Kevin. 2011. “Plate Tectonics, the Wilson Cycle, and Mantle Plumes: Geodynamics from the Top.” Annual Review of Earth and Planetary Sciences 39 (May): 1–29.
Campbell, Ian H. 2001. “Identification of Ancient Mantle Plumes.” In Mantle Plumes: Their Identification Through Time, edited by Richard E. Ernst, and Kenneth L. Buchan, 5–21. GSA Special Paper 352.
Campbell, Ian H. 2005. “Large Igneous Provinces and the Mantle Plume Hypothesis.” Elements 1, no. 5 (December): 265–269.
Campbell, Ian H. 2007. “Testing the Plume Theory.” Chemical Geology 241, nos. 3–4 (15 July): 153–176.
Campbell, Ian H., and Ross W. Griffiths. 1990. “Implications of Mantle Plume Structure For the Evolution of Flood Basalts.” Earth and Planetary Science Letters 99, nos. 1–2 (July): 79–93.
Cande, S. C., and D. V. Kent. 1995. “Revised Calibration of the Geomagnetic Polarity Timescale for the Late Cretaceous and Cenozoic.” Journal of Geophysical Research: Solid Earth 100, no. B4 (10 April): 6093–6095.
Cañón-Tapia, E. 2010. “Origin of Large Igneous Provinces: The Importance of a Definition.” In What is a Volcano? edited by Edgardo Cañón-Tapia, and Alexandru Szakács, 77–101. Geological Society of America Special Paper 470.
Cardoso, Tatiana R., and Antonio S. de Castro. 2005. “The Blackbody Radiation in a D-Dimensional Universe.” Revista Brasileira de Ensino de Física 27, no. 4: 559–563.
Cashman, Katharine V., and Marie Edmonds. 2019. “Mafic Glass Compositions: A Record of Magma Storage Conditions, Mixing and Ascent.” Philosophical Transactions of the Royal Society A 377, no. 2139 (25 February): 20180004. doi: 10.1098/rsta.2018.0004.
Cashman, Katharine V., R. Stephen J. Sparks, and Jonathan D. Blundy. 2017. “Vertically Extensive and Unstable Magmatic Systems: A Unified View of Igneous Processes.” Science 355, no. 6331 (24 March): eaag3055. doi: 10.1126/science.aag3055.
Cawood, Peter A., Chris J. Hawkesworth, Sergei A. Pisarevsky, Bruno Dhuime, Fabio A. Capitanio, and Oliver Nebel. 2018. “Geological Archive of the Onset of Plate Tectonics.” Philosophical Transactions of the Royal Society A 376, no. 2132 (13 November): 20170405. doi: 10.1098/rsta.2017.0405.
Chakraborty, S. 2006. “Diffusion Modeling as a Tool For Constraining Timescales of Evolution of Metamorphic Rocks.” Mineralogy and Petrology 88, nos. 1–2 (8 August): 7–27.
Chakraborty, Sumit. 2008. “Diffusion in Solid Silicates: A Tool to Track Timescales of Processes Comes of Age.” Annual Review of Earth and Planetary Sciences 36, no. 1 (30 May): 153–190.
Chambers, John E. 2004. “Planetary Accretion in the Inner Solar System.” Earth and Planetary Science Letters 223, nos. 3–4 (15 July): 241–252.
Chandler, Michael T., Paul Wessel, Brian Taylor, Maria Seton, Seung-Sep Kim, and Kiseong Hyeong. 2012. “Reconstructing Ontong Java Nui: Implications for Pacific Absolute Plate Motion, Hotspot Drift and True Polar Wander.” Earth and Planetary Science Letters 331–332 (15 May): 140–151.
Chen, Yongshun John. 1992. “Oceanic Crustal Thickness Versus Spreading Rate.” Geophysical Research Letters 19, no. 8 (24 April): 753–756.
Cho, Noah, John Baumgardner, Jesse Sherburn, and Mark Horstemeyer. 2018. “Numerical Investigation of Strength-Reducing Mechanisms of Mantle Rock During the Genesis Flood.” In Proceedings of the Eighth International Conference on Creationism, edited by J. H. Whitmore, 707–730. Pittsburgh, Pennsylvania: Creation Science Fellowship.
Clemens, J. D. 2005. “Granites and Granitic Magmas: Strange Phenomena and New Perspectives on Some Old Problems.” Proceedings of the Geologists’ Association 116, no. 1: 9–16.
Clemens, J. D., and C. K. Mawer. 1992. “Granitic Magma Transport by Fracture Propagation.” Tectonophysics 204, nos. 3–4 (15 April): 339–360.
Coffin, Millard F. and Olav Eldholm. eds. 1991. “Large Igneous Provinces: JOI/USSAC Workshop Report.” The University of Texas at Austin Institute for Geophysics Technical Report 114.
Coffin, Millard F., and Olav Eldholm. 1992. “Volcanism and Continental Break-Up: A Global Compilation of Large Igneous Provinces.” In Magmatism and the Causes of Continental Break-up, edited by B. C. Storey, T. Alabaster, and R. J. Pankhurst, 17–30. Geological Society of London Special Publication 68, no. 1.
Coffin, Millard F. and Olav Eldholm. 1994. “Large Igneous Provinces: Crustal Structure, Dimensions, and External Consequences.” Reviews of Geophysics 32, no. 1 (February): 1–36.
Coffin, Millard F., Robert A. Duncan, Olav Eldholm, J. Godfrey Fitton, Fred A. Frey, Hans Christian Larsen, John J. Mahoney et al. 2006. “Large Igneous Provinces and Scientific Ocean Drilling: Status Quo and a Look Ahead.” Oceanography 19, no. 4 (December): 150–160.
Coltice, N., B. R. Phillips, H. Bertrand, Y. Ricard, and P. Rey. 2007. “Global Warming of the Mantle at the Origin of Flood Basalts over Supercontinents.” Geology 35, no. 5 (May): 391–394.
Coltice, Nicolas, Hervé Bertrand, Patrice Rey, Fred Jourdan, Benjamin R. Phillips, and Yanick Ricard. 2009. “Global Warming of the Mantle Beneath Continents Back to the Archaean.” Gondwana Research 15, nos. 3–4 (June): 254–266.
Coltice, Nicolas, Mélanie Gérault, and Martina Ulvrová. 2017. “A Mantle Convection Perspective on Global Tectonics.” Earth-Science Reviews 165 (February): 120–150.
Coltice, Nicolas, Laurent Husson, Claudio Faccenna, and Maëlis Arnould. 2019. “What Drives Tectonic Plates?” Science Advances 5, no. 10 (30 October): eaax4295. doi: 10.1126/sciadv.aax4295.
Condie, Kent C., Richard C. Aster, and Jeroen van Hunen. 2016. “A Great Thermal Divergence in the Mantle Beginning 2.5 Ga: Geochemical Constraints From Greenstone Basalts and Komatiites.” Geoscience Frontiers 7, no. 4 (July): 543–553.
Condie, Kent C. 2018. “A Planet in Transition: The Onset of Plate Tectonics on Earth Between 3 and 2 Ga?” Geoscience Frontiers 9, no. 1 (January): 51–60.
Conrad, Clinton P., and Carolina Lithgow-Bertelloni. 2002. “How Mantle Slabs Drive Plate Tectonics.” Science 298, no. 5591 (4 October): 207–209.
Coogan, Laurence A., Gawen R. T. Jenkin, and Robert N. Wilson. 2002. “Constraining the Cooling Rate of the Lower Oceanic Crust: A New Approach Applied to the Oman Ophiolite.” Earth and Planetary Science Letters 199, nos. 1–2 (30 May): 127–146.
Coogan, L. A., G. R. T. Jenkin, and R. N. Wilson. 2007. “Contrasting Cooling Rates in the Lower Oceanic Crust at Fast- and Slow-Spreading Ridges Revealed by Geospeedometry.” Journal of Petrology 48, no. 11 (November): 2211–2231.
Coogan, L. A., A. D. Saunders, and R. N. Wilson. 2014. “Aluminum-in-Olivine Thermometry of Primitive Basalts: Evidence of an Anomalously Hot Mantle Source for Large Igneous Provinces.” Chemical Geology 368 (12 March): 1–10.
Cooper, Kari M. 2019. “Time Scales and Temperatures of Crystal Storage in Magma Reservoirs: Implications for Magma Reservoir Dynamics.” Philosophical Transactions of the Royal Society A 377, no. 2139 (25 February): 20180009. doi: 10.1098/rsta.2018.0009.
Costa, Fidel, Ralf Dohmen, and Sumit Chakraborty. 2008. “Time Scales of Magmatic Processes from Modeling the Zoning Patterns of Crystals.” Reviews in Mineralogy and Geochemistry 69, no. 1 (January 1): 545–594.
Costa, F., T. Shea, and T. Ubide. 2020. “Diffusion Chronometry and the Timescales of Magmatic Processes.” Nature Reviews Earth and Environment 1 (1 April): 201–214. doi: 10.1038/s43017-020-0038-x.
Costa, Fidel. 2021. “Clocks in Magmatic Rocks.” Annual Review of Earth and Planetary Sciences 49 (May): 231–252.
Coughenour, Christopher L., Allen W. Archer, and Kenneth J. Lacovara. 2009. “Tides, Tidalites, and Secular Changes in the Earth–Moon System.” Earth Science Reviews 97, nos. 1–4 (December): 59–79.
Courtillot, Vincent, Anne Davaille, Jean Besse, and Joann Stock. 2003. “Three Distinct Types of Hotspots in the Earth’s Mantle.” Earth and Planetary Science Letters 205, nos. 3–4 (10 January): 295–308.
Crameri, Fabio, Clinton P. Conrad, Laurent Montési, and Carolina R. Lithgow-Bertelloni. 2019. “The Dynamic Life of an Ocean Plate.” Tectonophysics 760 (5 June): 107–135.
Dalton, Colleen A., Charles H. Langmuir, and Allison Gale. 2014. “Geophysical and Geochemical Evidence for Deep Temperature Variations Beneath Mid-Ocean Ridges.” Science 344, no. 6179 (April 4): 80–83.
Dalton, Colleen A., Douglas S. Wilson, and Timothy D. Herbert. 2022. “Evidence for a Global Slowdown in Seafloor Spreading Since 15 Ma.” Geophysical Research Letters 49, no. 6 (28 March): e2022GL097937. doi: 10.1029/2022GL097937.
Davaille, Anne. 1999. “Simultaneous Generation of Hotspots and Superswells by Convection in a Heterogeneous Planetary Mantle.” Nature 402, no. 6763 (16 December): 756–760.
Davaille, Anne, and Barbara Romanowicz. 2020. “Deflating the LLSVPs: Bundles of Mantle Thermochemical Plumes Rather Than Thick Stagnant ‘Piles’.” Tectonics 39, no. 10 (October): e2020TC006265. doi: 10.1029/2020TC006265.
Davies, D. Rhodri, S. Goes, J. H. Davies, B. S. A. Schuberth, H.-P. Bunge, and J. Ritsema. 2012. “Reconciling Dynamic and Seismic Models of Earth’s Lower Mantle: The Dominant Role of Thermal Heterogeneity.” Earth and Planetary Science Letters 353–354 (1 November): 253–269.
Davies, D. Rhodri, S. Goes, and H. C. P. Lau. 2015. “Thermally Dominated Deep Mantle LLSVPs: A Review.” In The Earth’s Heterogeneous Mantle: A Geophysical, Geodynamical, and Geochemical Perspective, edited by Amir Khan, and Frédéric Deschamps, 441–477. Netherlands, Dordrecht: Springer.
DeGraff, James M., Philip E. Long, and Atilla Aydin. 1989. “Use of Joint-Growth Directions and Rock Textures to Infer Thermal Regimes During Solidification of Basaltic Lava Flows.” Journal of Volcanology and Geothermal Research 38, nos. 3–4 (September): 309–324.
Deschamps, Anne, Cécile Grigné, Morgane Le Saout, Samuel Adam Soule, Pascal Allemand, Brigitte Van Vliet-Lanoe, and France Floc’h. 2014. “Morphology and Dynamics of Inflated Subaqueous Basaltic Lava Flows.” Geochemistry, Geophysics, Geosystems 15, no. 6 (June): 2128–2150.
Dhuime, Bruno, Chris J. Hawkesworth, Peter A. Cawood, and Craig D. Storey. 2012. “A Change in the Geodynamics of Continental Growth 3 Billion Years Ago.” Science 335, no. 6074 (16 March): 1334–1336.
Doblas, Miguel, José López-Ruiz, José-María Cebriá, Nasrrddine Youbi, and Eugenio Degroote. 2002. “Mantle Insulation Beneath the West African Craton During the Precambrian-Cambrian Transition.” Geology 30, no. 9 (September 1): 839–842.
Dodson, Martin H. 1973. “Closure Temperature in Cooling Geochronological and Petrological Systems.” Contributions to Mineralogy and Petrology 40, no. 3 (September): 259–274.
Dodson, M. H. 1976. “Kinetic Processes and Thermal History of Slowly Cooling Solids.” Nature 259, no. 5544 (19 February): 551–553.
Drabon, Nadja, Benjamin L. Byerly, Gary R. Byerly, Joseph L. Wooden, Michael Wiedenbeck, John W. Valley, Kouki Kitajima, Ann M. Bauer, and Donald R. Lowe. 2022. “Destabilization of Long-Lived Hadean Protocrust and the Onset of Pervasive Hydrous Melting at 3.8 Ga.” AGU Advances 3, no. 2 (April): e2021AV000520. doi: 10.1029/2021AV000520.
Dziewonski, Adam M., and Don L. Anderson. 1981. “Preliminary Reference Earth Model.” Physics of the Earth and Planetary Interiors 25, no. 4 (June): 297–356.
Eakins, B. W., and G. F. Sharman. 2010. Volumes of the World’s Oceans from ETOPO1, NOAA National Geophysical Data Center, Boulder, Colorado. http://ngdc.noaa.gov/mgg/global/etopo1_ocean_volumes.html.
Echeverria, Lina M. 1980. “Tertiary or Mesozoic Komatiites from Gorgona Island, Colombia: Field Relations and Geochemistry.” Contributions to Mineralogy and Petrology 73, no. 3 (August): 253–266.
Edmonds, Marie, Katharine V. Cashman, Marian Holness, and Matthew Jackson. 2019. “Architecture and Dynamics of Magma Reservoirs.” Philosophical Transactions of the Royal Society A 377, no. 2139 (25 February): 20180298. doi: 10.1098/rsta.2018.0298.
Eldholm, Olav, and Millard F. Coffin. 2000. “Large Igneous Provinces and Plate Tectonics.” In: The History and Dynamics of Global Plate Motions, edited by Mark A. Richards, Richard G. Gordon, and Robert D. van der Hilst. American Geophysical Union Geophysical Monograph 121: 309–326.
El Dien, Hamed Gamal, Luc S. Doucet, J. Brendan Murphy, and Zheng-Xiang Li. 2020. “Geochemical Evidence for a Widespread Mantle Re-enrichment 3.2 Billion Years Ago: Implications for Global-Scale Plate Tectonics.” Scientific Reports 10: 9461. doi: 10.1038/s41598-020-66324-y.
Ernst, Richard E. 2007. “Mafic-Ultramafic Large Igneous Provinces (LIPs): Importance of the Pre-Mesozoic Record.” Episodes 30, no. 2 (June 1): 108–114.
Ernst, Richard E. 2016. “October 2016 LIP of the Month.” Global LIP Table 2.1 REV FINAL.XLSX. www.largeigneousprovinces.org/16oct.
Ernst, Richard E., and Kenneth L. Buchan. 1997. “Giant Radiating Dyke Swarms: Their Use in Identifying Pre-Mesozoic Large Igneous Provinces and Mantle Plumes.” In Large Igneous Provinces: Continental, Oceanic, and Planetary Flood Volcanism, edited by John J. Mahoney and Millard F. Coffin. Geophysical Monograph Series 100: 297–333.
Ernst, Richard E., Kenneth L. Buchan, and Ian H. Campbell. 2005. “Frontiers in Large Igneous Province Research.” Lithos 79, nos. 3–4 (February): 271–297.
Ernst, Richard E., David P. G. Bond, Shuan-Hong Zhang, Kenneth L. Buchan, Stephen E. Grasby, Nasrrddine Youbi, Hafida El Bilali, Andrey Bekker, and Luc S. Doucet. 2021. “Large Igneous Province Record Through Time and Implications for Secular Environmental Changes and Geological Time-Scale Boundaries.” In Large Igneous Provinces: A Driver of Global Environmental and Biotic Changes, edited by Richard E. Ernst, Alexander J. Dickson, and Andrey Bekker, 1–26. AGU Geophysical Monograph Series 255.
Ernst, Richard E., Wouter Bleeker, Ulf Söderlund, and Andrew C. Kerr. 2013. “Large Igneous Provinces and Supercontinents: Toward Completing the Plate Tectonic Revolution.” Lithos 174 (1 August): 1–14.
Ernst, W. G. 2017. “Earth’s Thermal Evolution, Mantle Convection, and Hadean Onset of Plate Tectonics.” Journal of Asian Earth Sciences 145B (1 September): 334–348.
Faak, Kathrin, Laurence A. Coogan, and Sumit Chakraborty. 2015. “Near Conductive Cooling Rates in the Upper-Plutonic Section of Crust Formed at the East Pacific.” Earth and Planetary Science Letters 423 (1 August): 36–47.
Faak, Kathrin, and Kathryn M. Gillis. 2016. “Slow Cooling of the Lowermost Oceanic Crust at the Fast-Spreading East Pacific Rise.” Geology 44, no. 2 (February): 115–118.
Faak, Kathi, Sumit Chakraborty, Laurence A. Coogan, and Ralf Dohmen. 2018. “Comment on ‘Formation of Fast-Spreading Lower Oceanic Crust as Revealed by a New Mg–REE Coupled Geospeedometer’ by Sun and Lissenberg.” Earth and Planetary Science Letters 502 (September): 284–286.
Falloon, Trevor J., Leonid V. Danyushevsky, Alexei Ariskin, David H. Green, and Clifford E. Ford. 2007. “The Application of Olivine Geothermometry to Infer Crystallization Temperatures of Parental Liquids: Implications For the Temperature of MORB Magmas.” Chemical Geology 241, nos. 3–4 (15 July): 207–233.
Fan, Qingkai, Jean-Arthur Olive, and Mathilde Cannat. 2021. “Thermo-Mechanical State of Ultraslow-Spreading Ridges With a Transient Magma Supply.” Journal of Geophysical Research: Solid Earth 126, no. 4 (April): e2020JB020557. doi: 10.1029/2020JB020557.
Faulkner, Danny R. 2013. “An Analysis of Astronomical Aspects of the Hydroplate Theory.” Creation Research Society Quarterly 49 (Winter): 197–210.
Fitton, J. Godfrey, John J. Mahoney, Paul J. Wallace, and Andrew D. Saunders. 2004. “Origin and Evolution of the Ontong Java Plateau: Introduction.” In Origin and Evolution of the Ontong Java Plateau, edited by J. G. Fitton, J. J. Mahoney, P. J. Wallace, and A. D. Saunders. Geological Society, London, Special Publications 229, no. 1 (January): 1–8.
Forbes, A. E. S., S. Blake, and H. Tuffen. 2014. “Entablature: Fracture Types and Mechanisms.” Bulletin of Volcanology 76, no. 5 (May): 820. doi: 10.1007/s00445-014-0820-z.
Ford, C. E., D. G. Russell, J. A. Craven, and M. R. Fisk. 1983. “Olivine-Liquid Equilibria: Temperature, Pressure and Composition Dependence of the Crystal/Liquid Cation Partition Coefficients for Mg, Fe2+, Ca and Mn.” Journal of Petrology 24, no. 3 (August): 256–266.
Forsyth, Donald, and Seiya Uyeda. 1975. “On the Relative Importance of the Driving Forces of Plate Motion.” Geophysical Journal of the Royal Astronomical Society 43, no. 1 (October): 163–200.
Foulger, Gillian R. 2012. “Are ‘Hot Spots’ Hot Spots?” Journal of Geodynamics 58, no. B12201 (July): 1–128.
Foulger, G. R. 2016. “Plates vs. Plumes: Lithosphere vs. Asthenosphere.” In Lithosphere 2016 (Ninth Symposium on the Structure, Composition and Evolution of the Lithosphere in Fennoscandia, edited by Ilmo Kukkonen, Suvi Heinonen, Kati Oinonen et al: 9–11. Helsinki, Finland: University of Helsinki.
Foulger, Gillian R., Tony Doré, C. Henry Emeleus, Dieter Franke, Laurent Geoffroy, Laurent Gernigon, Richard Hey, et al. 2020. “The Iceland Microcontinent and a Continental Greenland-Iceland-Faroe Ridge.” Earth-Science Reviews 206 (July): 102926. doi: 10.1016/j.earscirev.2019.102926.
Foulger, Gillian R., Christian Schiffer, and Alexander L. Peace. 2020. “A New Paradigm For the North Atlantic Realm.” Earth-Science Reviews 206 (July): 103038. doi: 10.1016/j.earscirev.2019.103038.
Frazier, Kendrick. 1969. “The New View of the World.” Science News 96, no. 19 (November 8): 430–433.
French, Scott W., and Barbara Romanowicz. 2015. “Broad Plumes Rooted At the Base of the Earth’s Mantle Beneath Major Hotspots.” Nature 525, no. 7567 (3 September): 95–99.
Frey, F. A., M. F. Coffin, P. J. Wallace, and D. Weis. 2003. “Leg 183 Synthesis: Kerguelen Plateau-Broken Ridge—A Large Igneous Province.” In Proceedings of the Ocean Drilling Programme, Scientific Results 183 (19 March), edited by F. A. Frey, M. F. Coffin, P. J. Wallace, and P. G. Quilty: 1–48. www-odp.tamu.edu/publications/183_sr/volume/synth/synth.pdf.
Frost, B. Ronald. 1991. “Introduction to Oxygen Fugacity and Its Petrologic Importance.” Reviews in Mineralogy and Geochemistry 25, no. 1 (January 1): 1–9.
Fundis, A. T., S. A. Soule, D. J. Fornari, and M. R. Perfit. 2010. “Paving the Seafloor: Volcanic Emplacement Processes During the 2005–2006 Eruptions At the Fast Spreading East Pacific Rise, 9°50′N.” Geochemistry, Geophysics, Geosystems 11, no. 8 (31 August): Q08024. doi: 10.1029/2010GC003058.
Furlong, Kevin P., and David S. Chapman. 2013. “Heat Flow, Heat Generation, and the Thermal State of the Lithosphere.” Annual Review of Earth and Planetary Sciences 41 (May): 385–410.
Ganne, Jérôme, and Xiaojun Feng. 2017. “Primary Magmas and Mantle Temperatures Through Time.” Geochemistry, Geophysics, Geosystems 18, no. 3 (March): 872–888.
Garnero, Edward J., Thorne Lay, and Allen McNamara. 2007. “Implications of Lower-Mantle Structural Heterogeneity for Existence and Nature of Whole-Mantle Plumes.” In Plate, Plumes and Planetary Processes, edited by Gillian R. Foulger, and Donna M. Jurdy, 79–101. Geological Society of America Special Paper 430.
Garnero, Edward J., Allen K. McNamara, and Sang-Heon Shim. 2016. “Continent-Sized Anomalous Zones With Low Seismic Velocity At the Base of Earth’s Mantle.” Nature Geoscience 9, no. 7 (July): 481–489.
Geng, Xianlei, Stephen F. Foley, Yongsheng Liu, Zaicong Wang, Zhaochu Wu, and Lian Zhou. 2019. “Thermal-Chemical Conditions of the North China Mesozoic Lithospheric Mantle and Implication For the Lithospheric Thinning of Cratons.” Earth and Planetary Science Letters 516 (15 June): 1–11.
Gernigon. L., D. Franke, L. Geoffroy, C. Schiffer, G. R. Foulger, and M. Stoker. 2020. “Crustal Fragmentation, Magmatism, and the Diachronous Opening of the Norwegian-Greenland Sea.” Earth-Science Reviews 206 (July): 102839. doi: 10.1016/j.earscirev.2019.04.011.
Gerya, Taras. 2019. “Geodynamics of the Early Earth: Quest For the Missing Paradigm.” Geology 47 (October 1): 1006–1007.
Gerya, T. V., R. J. Stern, M. Baes, S. V. Sobolev, and S. A. Whattam. 2015. “Plate Tectonics on the Earth Triggered by Plume-Induced Subduction Initiation.” Nature 527, no. 7577 (11 November): 221–225.
Gibson, S. A., R. N. Thompson, and J. A. Day. 2006. “Timescales and Mechanisms of Plume–Lithosphere Interactions: 40Ar/39Ar Geochronology and Geochemistry of Alkaline Igneous Rocks From the Paraná–Etendeka Large Igneous Province.” Earth and Planetary Science Letters 251, nos. 1–2 (15 November):1–17.
Gladczenko, Tadeusz P., Millard F. Coffin, and Olav Eldholm. 1997. “Crustal Structure of the Ontong Java Plateau: Modeling of New Gravity and Existing Seismic Data.” Journal of Geophysical Research 102, no. B10 (10 October): 22711–22729.
Glazner, Allen F. 2021. “Thermal Constraints on the Longevity, Depth, and Vertical Extent of Magmatic Systems.” Geochemistry, Geophysics, Geosystems 22, no. 4 (April) e2020GC009459. doi: 10.1029/2020GC009459.
Gordeychik, Boris, Tatiana Churikova, Andreas Kronz, Caren Sundermeyer, Alexander Simakin, and Gerhard Wörne. 2018. “Growth of, and Diffusion in, Olivine in Ultra-Fast Ascending Basalt Magmas From Shiveluch Volcano.” Scientific Reports 8: 11775. doi: 10.1038/s41598-018-30133-1.
Gorvett, Zaria. 2022. “Why Are There Continent-Sized ‘Blobs’ in the Deep Earth?” BBC Future (12 May). www.bbc.com/future/article/20220510-why-are-there-continent-sized-blobs-in-the-deep-earth?utm_source=pocket-newtab-global-en.
Gradstein, Felix M., James G. Ogg, and Frits J. Hilgen. 2012. “On the Geologic Time Scale.” Newsletters on Stratigraphy 45, no. 2 (April 1): 171–188.
Gramling, Carolyn. 2021. “How the Earth-Shaking Theory of Plate Tectonics Was Born.” Science News (16 January). https://www.sciencenews.org/article/earth-plate-tectonics-volcanoes-earthquakes-faults.
Greber, Nicolas D., Nicolas Dauphas, Andrey Bekker, Matouš P. Ptáček, Ilya N. Bindeman, and Axel Hofmann. 2017. “Titanium Isotopic Evidence for Felsic Crust and Plate Tectonics 3.5 Billion Years Ago.” Science 357, no. 6357 (September 22): 1271–1274.
Green, David H., Trevor J. Falloon, Stephen M. Eggins, and Gregory M. Yaxley. 2001. “Primary Magmas and Mantle Temperatures.” European Journal of Mineralogy 13, no. 3 (May 1): 437–452.
Gregg, Tracy K. P., and Jonathan H. Fink. 1995. “Quantification of Submarine Lava-Flow Morphology Through Analog Experiments.” Geology 23, no. 1 (January 1): 73–76.
Griffiths, Ross W., and Ian H. Campbell. 1990. “Stirring and Structure in Mantle Starting Plumes.” Earth and Planetary Science Letters 99, nos. 1–2 (July): 66–78.
Grose, Christopher J., and Juan C. Afonso. 2019. “New Constraints on the Thermal Conductivity of the Upper Mantle From Numerical Models of Radiation Transport.” Geochemistry, Geophysics, Geosystems 20, no. 5 (May): 2378–2394.
Grove, T. L., and S. W. Parman. 2004. “Thermal Evolution of the Earth as Recorded by Komatiites.” Earth and Planetary Science Letters 219, nos. 3–4 (15 March): 173–187.
Hallam, Tony. 2004. Catastrophes and Lesser Calamities: The Causes of Mass Extinctions. Oxford, United Kingdom: Oxford University Press.
Hamilton, Warren B. 2019. “Toward a Myth-Free Geodynamic History of Earth and its Neighbors.” Earth-Science Reviews 198 (November): 102905. doi: 10.1016/j.earscirev.2019.102905.
Hartlieb, P., M. Toifl, F. Kuchar, R. Meisels, and T. Antretter. 2016. “Thermo-Physical Properties of Selected Hard Rocks and Their Relation to Microwave-Assisted Comminution.” Minerals Engineering 91 (15 May): 34–41.
Hasterok, D. 2013. “A Heat Flow Based Cooling Model For Tectonic Plates.” Earth and Planetary Science Letters 361 (1 January): 34–43.
Hawkesworth, Chris J., Peter A. Cawood and Bruno Dhuime. 2020. “The Evolution of the Continental Crust and the Onset of Plate Tectonics.” Frontiers in Earth Science 8 (6 August): 326. doi: 10.3389/feart.2020.00326.
Hepworth, Luke N., J. Stephen Daly, Ralf Gertisser, Chris G. Johnson, C. Henry Emeleus, and Brian O’Driscoll. 2020. “Rapid Crystallization of Precious-Metal-Mineralized Layers in Mafic Magmatic Systems.” Nature Geoscience 13, no. 5 (May): 375–381.
Heron, Philip J., and Edward J. Garnero. 2019. “What Lies Beneath: Thoughts on the Lower Mantle.” Geoscientist 29, no. 3: 10–15. doi: 10.1144/geosci2019-015.
Herzberg, Claude. 1992. “Depth and Degree of Melting of Komatiites.” Journal of Geophysical Research: Solid Earth 97, no. B4 (10 April): 4521–4540.
Herzberg, Claude. 2004. “Geodynamic Information in Peridotite Petrology.” Journal of Petrology 45, no. 12 (December): 2507–2530.
Herzberg, C., and P. D. Asimow. 2015. “PRIMELT3 MEGA.XLSM Software for Primary Magma Calculation: Peridotite Primary Magma MgO Contents From the Liquidus to the Solidus.” Geochemistry, Geophysics, Geosystems 16, no. 2 (February): 563–578.
Herzberg, C., and P. D. Asimow. 2008. “Petrology of Some Oceanic Island Basalts: PRIMELT2.XLS Software For Primary Magma Calculation.” Geochemistry, Geophysics, Geosystems 9, no. 9 (September): Q09001. doi: 10.1029/2008GC002057.
Herzberg, C., P. D. Asimow, N. Arndt, Y. Niu, C. m. Lesher, J. G. Fitton, M. J. Cheadle, and A. D. Saunders. 2007. “Temperatures in Ambient Mantle and Plumes: Constraints From Basalts, Picrites, and Komatiites.” Geochemistry, Geophysics, Geosystems 8, no. 2 (February): Q02006. doi: 10.1029/2006GC001390.
Herzberg, Claude, Kent Condie, and Jun Korenaga. 2010. “Thermal History of the Earth and Its Petrological Expression.” Earth and Planetary Science Letters 292, nos. 1–2 (15 March): 79–88.
Herzberg, Claude, and Esteban Gazel. 2009. “Petrological Evidence for Secular Cooling in Mantle Plumes.” Nature 458, no. 7238 (2 April): 619–622.
Herzberg, C., and M. J. O’Hara. 2002. “Plume-Associated Ultramafic Magmas of Phanerozoic Age.” Journal of Petrology 43, no. 1 (October): 1857–1883. Herzberg, Claude. 2011. “Basalts as Temperature Probes of Earth’s Mantle.” Geology 39, no. 12 (December 1): 1179–1180.
Ho, Anita M., and Katharine V. Cashman. 1997. “Temperature Constraints on the Ginkgo Flow of the Columbia River Basalt Group.” Geology 25, no. 5 (May 1): 403–406.
Hoernle, Kaj, Folkmar Hauff, Paul van den Bogaard, Reinhard Werner, Nick Mortimer, Jörg Geldmacher, Dieter Garbe-Schönberg, and Bryan Davy. 2010. “Age and Geochemistry of Volcanic Rocks From the Hikurangi and Manihiki Oceanic Plateaus.” Geochimica et Cosmochimica Acta 74, no. 24 (15 December): 7196–7219.
Hole, Malcolm J., and James H. Natland. 2020. “Magmatism in the North Atlantic Igneous Province; Mantle Temperatures, Rifting and Geodynamics.” Earth-Science Reviews 206 (July): 102794. doi: 10.1016/j.earscirev.2019.02.011.
Holmes, Arthur. 1931. “Radioactivity and Earth Movements.” Transactions of the Geological Society of Glasgow 18: 559–606. doi: 10.1144/transglas.18.3.559.
Hon, Ken, Jim Kauahikaua, Roger Denlinger, and Kevin Mackay. 1994. “Emplacement and Inflation of Pahoehoe Sheet Flows: Observations and Measurements of Active Lava Flows on Kilauea Volcano, Hawaii.” Geological Society of America Bulletin 106, no. 3 (March 1): 351–370.
Hopkins, Michelle, T. Mark Harrison, and Craig E. Manning. 2008. “Low Heat Flow Inferred from >4 Gyr Zircons Suggests Hadean Plate Boundary Interactions.” Nature 456, no. 7221 (27 November): 493–496.
Hopkins, Michelle T., T. Mark Harrison, and Craig E. Manning. 2010. “Constraints on Hadean Geodynamics from Mineral Inclusions in >4 Ga Zircons.” Earth and Planetary Science Letters 298, nos. 3–4 (1 October): 367–376.
Huang, Hsin-Hua, Fan-Chi Lin, Brandon Schmandt, Jamie Farrell, Robert B. Smith, and Victor C. Tsai. 2015. “The Yellowstone Magmatic System from the Mantle Plume to the Upper Crust.” Science 348, no. 6236 (23 April): 773–776.
Humphreys, D. Russell. 2000. “Accelerated Nuclear Decay: A Viable Hypothesis?” In Radioisotopes and the Age of the Earth: A Young-Earth Creationist Research Initiative, edited by Larry Vardiman, Andrew A. Snelling, and Eugene F. Chaffin, 333–379. El Cajon, California: Institute for Creation Research and St. Joseph, Missouri: Creation Research Society.
Humphreys, D. Russell. 2005. “Young Helium Diffusion Age of Zircons Supports Accelerated Nuclear Decay. In Radioisotopes and the Age of the Earth: Results of A Young-Earth Creationist Research Initiative, edited by Larry Vardiman, Andrew A. Snelling, and Eugene F. Chaffin, 25–100. El Cajon, California: Institute for Creation Research and St. Joseph, Missouri: Creation Research Society.
Humphreys, D. R. 2022. Personal communication, 4 May, 2022.
Humphreys, Russell. 2018. “New Mechanism for Accelerated Removal of Excess Radiogenic Heat.” In Proceedings of the Eighth International Conference on Creationism, edited by J. H. Whitmore, 731–739. Pittsburgh, Pennsylvania: Creation Science Fellowship. doi: 10.15385/jpicc.2018.8.1.49.
Ishii, Miaki, and Jeroen Tromp. 1999. “Normal-Mode and Free-Air Gravity Constraints on Lateral Variations in Velocity and Density of Earth’s Mantle.” Science 285, no. 5431 (20 August): 1231–1236.
Isley, Ann E., and Dallas H. Abbott. 1999. “Plume-Related Mafic Volcanism and the Deposition of Banded Iron Formation.” Journal of Geophysical Research: Solid Earth 104, no. B7 (10 July): 15461–15477.
Isley, Ann E., and Dallas H. Abbott. 2002. “Implications of the Temporal Distribution of High-Mg Magmas for Mantle Plume Volcanism Through Time.” Journal of Geology 110, no. 2 (March): 141–158.
Jackson, M. D., J. Blundy, and R. S. J. Sparks. 2018. “Chemical Differentiation, Cold Storage and Remobilization of Magma in the Earth’s Crust.” Nature 564, no. 7736 (3 December): 405–409.
Karakas, Ozge, Wim Degruyter, Olivier Bachmann, and Josef Dufek. 2017. “Lifetime and Size of Shallow Magma Bodies Controlled by Crustal-Scale Magmatism.” Nature Geoscience 10, no. 6 (29 May): 446–450.
Keller, C. Brenhin, and Blair Schoene. 2012. “Statistical Geochemistry Reveals Disruption in Secular Lithospheric Evolution About 2.5 Gyr Ago.” Nature 485, no. 7399 (24 May): 490–453.
Kellogg, L. H., and G. J. Wasserburg. 1990. “The Role of Plumes in Mantle Helium Fluxes.” Earth and Planetary Science Letters 99, no. 3 (August): 276–289.
Kerr, Andrew C., and John J. Mahoney. 2007. “Oceanic Plateaus: Problematic Plumes, Potential Paradigms.” Chemical Geology 241, nos. 3–4 (15 July): 332–353.
Kirkland, C. L., M. I. H. Hartnady, M. Barham, H. K. H. Olierook, A. Steenfelt, and J. A. Hollis. 2021. “Widespread Reworking of Hadean-to-Eoarchean Continents During Earth’s Thermal Peak.” Nature Communications 12 (12 January): 331. doi: 10.1038/s41467-020-20514-4.
Klein, Emily M., and Charles H. Langmuir. 1987. “Global Correlations of Ocean Ridge Basalt Chemistry With Axial Depth and Crustal Thickness.” Journal of Geophysical Research Solid Earth 92, no. B8 (10 July): 8089–8115.
Koelemeijer, Paula, Arwen Deuss, and Jeroen Ritsema. 2017. “Density Structure of Earth’s Lowermost Mantle from Stoneley Mode Splitting Observations.” Nature Communications 8 (15 May): 15241. doi: 10.1038/ncomms15241.
Koepke, J., D. Garbe-Schönberg , T. Müller, D. Mock, S. Müller, and S. Nasir. 2022. “A Reference Section Through Fast-Spread Lower Oceanic Crust, Wadi Gideah, Samail Ophiolite (Sultanate of Oman): Petrography and Petrology.” Journal of Geophysical Research: Solid Earth 127, no. 1 (January): e2021JB022735. doi: 10.1029/2021JB022735.
Kopp, Greg, and Judith L. Lean. 2011. “A New, Lower Value of Total Solar Irradiance: Evidence and Climate Significance.” Geophysical Research Letters 38, no. 1 (16 January) L01706. doi: 10.1029/2010GL045777.
Korenaga, Jun. 2005. “Why Did Not the Ontong Java Plateau Form Subaerially?” Earth and Planetary Science Letters 234, nos. 3–4 (15 June): 385–399.
Korenaga, Jun. 2008a. “Plate Tectonics, Flood Basalts and the Evolution of the Earth’s Oceans.” Terra Nova 20, no. 6 (December): 419–439.
Korenaga, Jun. 2008b. “Urey Ratio and the Structure and Evolution of Earth’s Mantle.” Reviews of Geophysics 46, no. 2 (June): RG2007. doi: 10.1029/2007RG000241.
Korenaga, Jun. 2010. “Scaling of Plate-Tectonic Convection with Pseudoplastic Rheology.” Journal of Geophysical Research 115, no. B11405. doi.org/10.1029/2010JB007670.
Korenaga, Jun. 2013. “Initiation and Evolution of Plate Tectonics on Earth: Theories and Observations.” Annual Review of Earth and Planetary Science 41: 117–151.
Korenaga, Jun. 2018. “Crustal Evolution and Mantle Dynamics Through Earth History.” Philosophical Transactions of the Royal Society A: Mathematical, Physical and Engineering Sciences 376, no. 2132 (13 November): 20170408. doi: 10.1098/rsta.2017.0408.
Korneeva, Alina A., Nikolai Nekrylov, Vadim S. Kamenetsky, Maxim V. Portnyagin, Dmitry P. Savelyev, Stepan P. Krasheninnikov, Adam Abersteiner, Maya B. Kamenetsky, Michael E. Zelenski, Vasily D. Shcherbakov, and Roman E. Botcharnikov. 2020. “Composition, Crystallization Conditions and Genesis of Sulfide-Saturated Parental Melts of Olivine-Phyric Rocks from Kamchatsky Mys (Kamchatka, Russia).” Lithos 370–371 (October): 105657. doi: 10.1016/j.lithos.2020.105657.
Kusky, Timothy M., Brian F. Windley, and Ali Polat. 2018. “Geological Evidence For the Operation of Plate Tectonics Throughout the Archean: Records from Archean Paleo-Plate Boundaries.” Journal of Earth Science 29, no. 6 (19 December): 1291–1303. doi: 10.1007/s12583-018-0999-6.
Larsen, Tine B., and David A. Yuen. 1997. “Fast Plumeheads: Temperature-Dependent Versus Non-Newtonian Rheology.” Geophysical Research Letters 24, no. 16 (15 August): 1995–1998.
Lau, Harriet C. P., Jerry X. Mitrovica, James L. Davis, Jeroen Tromp, Hsin-Ying Yang, and David Al-Attar. 2017. “Tidal Tomography Constrains Earth’s Deep-Mantle Buoyancy.” Nature 551, no. 7680 (16 November): 321–326.
Le Bas, M. J., and A. L. Streckeisen. 1991. “The IUGS Systematics of Igneous Rocks.” Journal of the Geological Society 148, no. 5 (September): 825–833.
Lekic, Vedran, Sanne Cottaar, Adam Dziewonski, and Barbara Romanowicz. 2012. “Cluster Analysis of Global Lower Mantle Tomography: A New Class of Structure and Implications for Chemical Heterogeneity.” Earth and Planetary Science Letters 357–358 (1 December): 68–77.
Le Maitre, R. W., A. Streckeisen, B. Zanettin, M. J. Le Bas, B. Bonin, P. Bateman, G. Bellieni, et al. (eds). 2002. Igneous Rocks: A Classification and Glossary of Terms: Recommendations of the International Union of Geological Sciences Subcommission on the Systematics of Igneous Rocks. 2nd ed. Cambridge, United Kingdom: Cambridge University Press.
Lenardic, Adrian. 2017. “A Supercontinental Boost.” Nature Geoscience 10: 4–5. doi: 10.1038/ngeo2862.
Lenardic, A., L. Moresi, A. m. Jellinek, C. J. O’Neill, C. m. Cooper, and C. T. Lee. 2011. “Continents, Supercontinents, Mantle Thermal Mixing, and Mantle Thermal Isolation: Theory, Numerical Simulations, and Laboratory Experiments.” Geochemistry, Geophysics, Geosystems 12, no. 10 (October): Q10016. doi: 10.1029/2011GC003663.
Lesher, Charles E., and Frank J. Spera. 2015. “Thermodynamic and Transport Properties of Silicate Melts and Magma.” In The Encyclopedia of Volcanoes, 2nd ed. edited by Haraldur Sigurdsson, Bruce Houghton, Steve McNutt, Hazel Rymer, and John Stix, 113–141. Cambridge, United Kingdom: Academic Press.
Lipman, Peter W., and Olivier Bachmann. 2015. “Ignimbrites to Batholiths: Integrating Perspectives from Geological, Geophysical, and Geochronological Data.” Geosphere 11, no. 3 (June 1): 705–743.
Liu, He, Wei-dong Sun, Robert Zartman, and Ming Tang. 2019. “Continuous Plate Subduction Marked By the Rise of Alkali Magmatism 2.1 Billion Years Ago.” Nature Communications 10 (30 July): 3408. doi: 10.1038/s41467-019-11329-z.
Long, Philip E., and Bernard J. Wood. 1986. “Structures, Textures, and Cooling Histories of Columbia River Basalt Flows.” Geological Society of America Bulletin 97, no. 9 (September 1): 1144–1155.
Maher, Sarah M., Jeffrey S. Gee, Michael J. Cheadle, and Barbara E. John. 2021. “Three-Dimensional Magnetic Stripes Require Slow Cooling in Fast-Spread Lower Ocean Crust.” Nature 597, no. 7877 (22 September): 511–515.
Maher, S. m., J. S. Gee, A. K. Doran, M. J. Cheadle, and B. E. John. 2020. “Magnetic Structure of Fast-Spread Oceanic Crust at Pito Deep.” Geochemistry, Geophysics, Geosystems 21, no. 2 (February): 1–18.
Mahoney, J. J., J. G. Fitton, P. J. Wallace, and the Leg 192 Scientific Party. 2001. Proceedings of the Ocean Drilling Program, Initial Reports. Vol. 192. 1. Summary. College Station, Texas. doi: 10.2973/odp.proc.ir.192.2001.
Mahoney, J. J., M. Storey, R. A. Duncan, K. J. Spencer, and M. Pringle. 1993. “Geochemistry and Age of the Ontong Java Plateau.” In The Mesozoic Pacific: Geology, Tectonics, and Volcanism, edited by Malcolm S. Pringle, William W. Sager, William V. Sliter, and Seth Stein, 233–262. American Geophysical Union Monograph vol. 77 (1 January): 233– 262.
Mallmann, Guilherme, and Hugh St. C. O’Neill. 2013. “Calibration of an Empirical Thermometer and Oxybarometer Based on the Partitioning of Sc, Y and V Between Olivine and Silicate Melt.” Journal of Petrology 54, no. 5 (May): 933–945.
Marignier, Augustin, Ana M. G. Ferreira, and Thomas Kitching. 2020. “The Probability of Mantle Plumes in Global Tomographic Models.” Geochemistry, Geophysics, Geosystems 21: e2020GC009276. doi: 10.1029/2020GC009276.
Maruyama, Shigenori, M. Santosh, and Shintaro Azuma. 2018. “Initiation of Plate Tectonics in the Hadean: Eclogitization Triggered by the ABEL Bombardment.” Geoscience Frontiers 9, no. 4 (July): 1033–1048.
Marzoli, Andrea, Paul R. Renne, Enzo M. Piccirillo, Marcia Ernesto, Giuliano Bellieni, and Angelo De Min. 1999. “Extensive 200-Million-Year-Old Continental Flood Basalts of the Central Atlantic Magmatic Province.” Science 284, no. 5414 (23 April): 616–618.
Mason, Ben G., David M. Pyle, and Clive Oppenheimer. 2004. “The Size and Frequency of the Largest Explosive Eruptions on Earth.” Bulletin of Volcanology 66, no. 8 (December): 735–748.
Matthews, S., O. Shorttle, and John Maclennan. 2016. “The Temperature of the Icelandic Mantle from Olivine-Spinel Aluminum Exchange Thermometry.” Geochemistry, Geophysics, Geosystems 17, no. 11 (November): 4725–4752.
McCaffrey, K. J. W., and N. Petford. 1997. “Are Granitic Intrusions Scale Invariant?” Journal of the Geological Society 154, no. 1 (February): 1–4.
McClain, James S., and Carl A. Atallah. 1986. “Thickening of the Oceanic Crust with Age.” Geology 14, no. 7 (July 1): 574–576.
McKenzie, D., and M. J. Bickle. 1988. “The Volume and Composition of Melt Generated by Extension of the Lithosphere.” Journal of Petrology 29, no. 3 (June): 625–679.
McNamara, Allen K., and Shijie Zhong. 2005. “Thermochemical Structures Beneath Africa and the Pacific Ocean.” Nature 437, no. 7062 (20 October): 1136–1139.
Milner, S. C., A. R. Duncan, and A. Ewart. 1992. “Quartz Latite Rheoignimbrite Flows of the Etendeka Formation, North-Western Namibia.” Bulletin of Volcanology 54, no. 3 (February): 200–219.
Mittal, Tushar, and Mark A. Richards. 2021. “The Magmatic Architecture of Continental Flood Basalts: 2. A New Conceptual Model.” Journal of Geophysical Research: Solid Earth 126, no. 12 (December): e2021JB021807. doi: 10.1029/2021JB021807.
Mittal, Tushar, Mark A. Richards, and Isabel M. Fendley. 2021. “The Magmatic Architecture of Continental Flood Basalts I: Observations From the Deccan Traps.” Journal of Geophysical Research: Solid Earth 126, no. 12 (December): e2021JB021808. doi: 10.1029/2021JB021808.
Montelli, R., G. Nolet, F. A. Dahlen, and G. Masters. 2006. “A Catalogue of Deep Mantle Plumes: New Results from Finite-Frequency Tomography.” Geochemistry, Geophysics, Geosystems 7, no. 11 (November): Q11007. doi: 10.1029/2006GC001248.
Montelli, Raffaella, Guust Nolet, F. A. Dahlen, Guy Masters, E. Robert Engdahl, and Shu-Huei Hung. 2004. “Finite-Frequency Tomography Reveals a Variety of Plumes in the Mantle.” Science 303, no. 5656 (16 January): 338–343.
Moon, Vicki G. 1993. “Geotechnical Characteristics of Ignimbrite: A Soft Pyroclastic Rock Type.” Engineering Geology 35, nos. 1–2 (October): 33–48.
Morgan, D. J., S. Blake, N. W. Rogers, B. DeVivo, G. Rolandi, R. Macdonald, and C. J. Hawkesworth. 2004. “Time Scales of Crystal Residence and Magma Chamber Volume From Modelling of Diffusion Profiles in Phenocrysts: Vesuvius 1944.” Earth and Planetary Science Letters 222, nos. 3–4 (15 June): 933–946.
Morgan, W. J. 1971. “Convection Plumes in the Lower Mantle.” Nature 230, no. 5288 (5 March): 42–43.
Morgan, W. Jason. 1972. “Deep Mantle Convection Plumes and Plate Motions.” American Association of Petroleum Geologists Bulletin 56, no. 2 (February 1): 203–213.
Müller, R. Dietmar, Walter R. Roest, Jean-Yves Royer, Lisa M. Gahagan, and John G. Sclater. 1997. “Digital Isochrons of the World’s Ocean Floor.” Journal of Geophysical Research: Solid Earth 102, no. B2 (10 February): 3211–3214.
Müller, R. Dietmar, Maria Sdrolias, Carmen Gaina, and Walter R. Roest. 2008. “Age, Spreading Rates, and Spreading Asymmetry of the World’s Ocean Crust.” Geochemistry, Geophysics, Geosystems 9, no. 4 (April): Q04006. doi: 10.1029/2007GC001743.
Müller, Samuel, Dieter Garbe-Schönberg, Jürgen Koepke, and Kaj Hoernle. 2022. “A Reference Section Through Fast-Spread Lower Oceanic Crust, Wadi Gideah, Samail Ophiolite (Sultanate of Oman): Trace Element Systematics and REE Crystallization Temperatures—Implications for Hybrid Crustal Accretion.” Journal of Geophysical Research: Solid Earth 127, no. 3 (March): e2021JB022699. doi: 10.1029/2021JB022699.
Navarro, Evan Arthur. 2021. “A Catastrophic Plate Tectonics Explanation for Earth’s Large Low Shear Velocity Provinces (Also Known as Superplumes).” Creation Research Society Quarterly 57, no. 3 (Winter): 168–174.
Nayar, Kishor G., Mostafa H. Sharqawy, Leonardo D. Banchik, and John H. Lienhard V. 2016. “Thermophysical Properties of Seawater: A Review and New Correlations That Include Pressure Dependence.” Desalination 390 (15 July): 1–24.
Neal, Clive R., Millard F. Coffin, and William W. Sager. 2019. “Contributions of Scientific Ocean Drilling to Understanding the Emplacement of Submarine Large Igneous Provinces and Their Effects on the Environment.” Oceanography 32, no. 1 (March 18): 176–192.
Ni, Sidao, Eh Tan, Michael Gurnis, and Don Helmberger. 2002. “Sharp Sides to the African Superplume.” Science 296, no. 5574 (7 June): 1850–1852.
Nisbet, E. G., M. J. Cheadle, N. T. Arndt, and M. J. Bickle. 1993. “Constraining the Potential Temperature of the Archaean Mantle: A Review of the Evidence From Komatiites.” Lithos 30, nos. 3–4 (September): 291–307.
Niu, Yaoling. 2018. “Origin of the LLSVPs at the Base of the Mantle is a Consequence of Plate Tectonics—A Petrological and Geochemical Perspective.” Geoscience Frontiers 9, no. 5 (September): 1265–1278.
Niu, Yaoling. 2020. “On the Cause of Continental Breakup: A Simple Analysis in Terms of Driving Mechanisms of Plate Tectonics and Mantle Plumes.” Journal of Asian Earth Sciences 194 (1 June): 104367. doi: 10.1016/j.jseaes.2020.104367.
Niu, Yaoling. 2021. “Lithosphere Thickness Controls the Extent of Mantle Melting, Depth of Melt Extraction and Basalt Compositions in all Tectonic Settings on Earth—A Review and New Perspectives.” Earth-Science Reviews 217 (June): 103614. doi: 10.1016/j.earscirev.2021.103614.
Oard, Michael J. 1990. An Ice Age Caused by the Genesis Flood. El Cajon, California: Institute for Creation Research.
Oard, Michael J. 1999. “Very Rapid Emplacement of Columbia River Basalts in Non-Turbulent Flow.” CEN Technical Journal 13, no. 2 (November): 8–9.
Oard, Michael J. 2013. “Analysis of Walt Brown’s Flood Model.” https://creation.com/hydroplate-theory.
Palin, Richard M., M. Santosh, Wentao Cao, Shan-Shan Li, David Hernández-Uribe, and Andrew Parsons. 2020. “Secular Change and the Onset of Plate Tectonics on Earth.” Earth-Science Reviews 207 (August): 103172. doi: 10.1016/j.earscirev.2020.103172.
Parsons, Barry. and John G. Sclater. 1977. “An Analysis of the Variation of Ocean Floor Bathymetry and Heat Flow with Age.” Journal of Geophysical Research 82, no. 5 (10 February): 803–827.
Pastor-Galán, Daniel, R. Damian Nance, J. Brendan Murphy and Christopher J. Spencer. 2018. “Supercontinents: Myths, Mysteries, and Milestones.” In Fifty Years of the Wilson Cycle Concept in Plate Tectonics, edited by R. W. Wilson, G. A. Houseman, K. J. W. McCaffrey, A. G. Doré, and S. J. H. Buiter, 39–64. Geological Society of London Special Publication 470.
Peate, David W. 1997. “The Paraná-Etendeka Province.” In Large Igneous Provinces: Continental, Oceanic, and Planetary Flood Volcanism, edited by John J. Mahoney and Millard F. Coffin, 217–245. AGU Geophysical Monograph Series 100.
Petford, N., A. R. Cruden, K. J. W. McCaffrey, and J.-L. Vigneresse. 2000. “Granite Magma Formation, Transport and Emplacement in the Earth’s Crust.” Nature 408, no. 6813 (7 December): 669–673.
Petford, Nick, Ross C. Kerr, and John R. Lister. 1993. “Dike Transport of Granitoid Magmas.” Geology 21, no. 9 (September): 845–848.
Phillips, Benjamin R., and Nicolas Coltice. 2010. “Temperature Beneath Continents as a Function of Continental Cover and Convective Wavelength.” Journal of Geophysical Research: Solid Earth 115, no. B4 (April): B04408. doi: 10.1029/2009JB006600.
Phipps Morgan, Jason, and Y. John Chen. 1993. “The Genesis of Oceanic Crust: Magma Injection, Hydrothermal Circulation, and Crustal Flow.” Journal of Geophysical Research: Solid Earth 98, no. B4 (10 April): 6283–6297.
Presnall, Dean C., and Gudmundur H. Gudfinnsson. 2011. “Oceanic Volcanism From the Low-Velocity Zone—Without Mantle Plumes.” Journal of Petrology 52, nos. 7–8 (July–August): 1533–1546.
Putirka, Keith D. 2005. “Mantle Potential Temperatures at Hawaii, Iceland, and the Mid-Ocean Ridge System, as Inferred From Olivine Phenocrysts: Evidence for Thermally Driven Mantle Plumes.” Geochemistry, Geophysics, Geosystems 6, no. 5 (May): Q05L08. doi: 10.1029/2005GC000915.
Putirka, Keith D., Michael Perfit, F. J. Ryerson, and Matthew G. Jackson. 2007. “Ambient and Excess Mantle Temperatures, Olivine Thermometry and Active vs. Passive Upwelling.” Chemical Geology 241, nos. 3–4 (15 July): 177–206.
Rees, Martin. 1999. Just Six Numbers: The Deep Forces That Shape The Universe. Burlington, Vermont: Phoenix.
Reidel, Stephen P. 1998. “Emplacement of Columbia River Flood Basalt.” Journal of Geophysical Research 103, no. B11 (November 10): 27393–27410.
Reidel, Stephen Paul. 2015. “Igneous Rock Associations 15. The Columbia River Basalt Group: A Flood Basalt Province in the Pacific Northwest, USA.” Geoscience Canada 42, no. 1 (February): 151–168.
Richards, Mark A., Robert A. Duncan, and Vincent E. Courtillot. 1989. “Flood Basalts and Hot-Spot Tracks: Plume Heads and Tails.” Science 246, no. 4926 (6 October): 103–107.
Richardson, W. Philip, Emile A. Okal, and Suzan Van der Lee. 2000. “Rayleigh–wave Tomography of the Ontong–Java Plateau.” Physics of the Earth and Planetary Interiors 118, nos. 1–2 (February): 29–51.
Roberge, Julie, Paul J. Wallace, Rosalind V. White and Millard F. Coffin. 2005. “Anomalous Uplift and Subsidence of the Ontong Java Plateau Inferred From CO2 Contents of Submarine Basaltic Glasses.” Geology 33, no. 6 (June 1): 501–504.
Robinson, A. H., L. Zhang, R. W. Hobbs, C. Peirce, and V. C. H. Tong. 2020. “Magmatic and Tectonic Segmentation of the Intermediate-Spreading Costa Rica Rift—A Fine Balance Between Magma Supply Rate, Faulting and Hydrothermal Circulation.” Geophysical Journal International 222, no. 1 (July): 132–152.
Roeder, P. L., and R. F. Emslie. 1970. “Olivine-Liquid Equilibrium.” Contributions to Mineralogy and Petrology 29, no. 4 (December): 275–289.
Rolf, T., N. Coltice, and P. J. Tackley. 2012. “Linking Continental Drift, Plate Tectonics and the Thermal State of the Earth’s Mantle.” Earth and Planetary Science Letters 351–352 (15 October): 134–146.
Rubin, Allison E., Kari M. Cooper, Christy B. Till, Adam J. R. Kent, Fidel Costa, Maitrayee Bose, Darren Gravley, Chad Deering, and Jim Cole. 2017. “Rapid Cooling and Cold Storage in a Silicic Magma Reservoir Recorded in Individual Crystals.” Science 356, no. 6343 (16 June): 1154–1156.
Rubin, Kenneth H., S. Adam Soule, William W. Chadwick Jr., Daniel J. Fornari, Daniel A. Clague, Robert W. Embley, Edward T. Baker, Michael R. Perfit, David W. Caress, and Robert P. Dziak. 2012. “Volcanic Eruptions in the Deep Sea.” Oceanography 25, no. 1 (March): 142–157.
Sarafian, Emily, Glenn A. Gaetani, Erik H. Hauri, and Adam R. Sarafian. 2017. “Experimental Constraints on the Damp Peridotite Solidus and Oceanic Mantle Potential Temperature.” Science 355, no. 6328 (3 March): 942–945.
Schmitt, Axel K. 2011. “Uranium Series Accessory Crystal Dating of Magmatic Processes.” Annual Review of Earth and Planetary Sciences 39 (May): 321–349.
Schubert, G., G. Masters, P. Olson, and P. Tackley. 2004. “Superplumes or Plume Clusters?” Physics of the Earth and Planetary Interiors 146, nos. 1–2 (16 August): 147–162.
Schuberth, B. S. A., H.-P. Bunge, and J. Ritsema. 2009. “Tomographic Filtering of High-Resolution Mantle Circulation Models: Can Seismic Heterogeneity be Explained by Temperature Alone?” Geochemistry, Geophysics, Geosystems 10, no. 5 (May): Q01W03. doi: 10.1029/2009GC002401.
Schwartz, Joshua J., Barbara E. John, Michael J. Cheadle, Peter W. Reiners, and A. Graham Baines. 2009. “Cooling History of Atlantis Bank Oceanic Core Complex: Evidence for Hydrothermal Activity 2.6 Ma off Axis.” Geochemistry, Geophysics, Geosystems 10, no. 8 (August): Q08020. doi: 10.1029/2009GC002466.
Self, S., L. Keszthelyi, and Th. Thordarson. 1998. “The Importance of Pāhoehoe.” Annual Review of Earth and Planetary Sciences 26: (May) 81–110.
Self, S., A. E. Jay, M. Widdowson, and L. P. Keszthelyi. 2008. “Correlation of the Deccan and Rajahmundry Trap Lavas: Are These the Longest and Largest Lava Flows on Earth?” Journal of Volcanology and Geothermal Research 172, nos. 1–2 (10 May): 3–19.
Self, S., A. Schmidt, and T. A. Mather. 2014. “Emplacement Characteristics, Time Scales, and Volcanic Gas Release Rates of Continental Flood Basalt Eruptions on Earth.” In Volcanism, Impacts, and Mass Extinctions: Causes and Effects, edited by Gerta Keller and Andrew C. Kerr, 319–338. Geological Society of America Special Paper 505.
Self, Stephen, Tushar Mittal, and Anne Elizabeth Jay. 2021. “Thickness Characteristics of Pāhoehoe Lavas in the Deccan Province, Western Ghats, India, and in continental flood basalt provinces elsewhere. Frontiers in Earth Science 8:630604 [doi: 10.3389/feart.2020.630604].
Seton, M., R. D. Müller, S. Zahirovic, C. Gaina, T. Torsvik, G. Shephard, A. Talsma, M. Gurnis, M. Turner, S. Maus, and M. Chandler. 2012. “Global Continental and Ocean Basin Reconstructions Since 200 Ma.” Earth Science Reviews 113, nos. 3–4 (July): 212–270.
Sharqawy, Mostafa H., John H. Lienhard V, and Syed M. Zubair. 2010. “Thermophysical Properties of Seawater: A Review of Existing Correlations and Data.” Desalination and Water Treatment 16, nos. 1–3 (April): 354–380.
Shea, Thomas, Kendra J. Lynn, and Michael O. Garcia. 2015. “Cracking the Olivine Zoning Code: Distinguishing Between Crystal Growth and Diffusion.” Geology 43, no. (October): 935–938.
Sheth, Hetu C. 2007. “‘Large Igneous Provinces (LIPs)’: Definition, Recommended Terminology and a Hierarchical Classification.” Earth-Science Reviews 85, nos. 3–4 (December): 117–124.
Silver, Paul G., Mark D. Behn, Katherine Kelley, Mark Schmitz, and Brian Savage. 2006. “Understanding Cratonic Flood Basalts.” Earth and Planetary Science Letters 245, nos. 1–2 (15 May): 190–201.
Sleep, Norman H. 1990. “Hotspots and Mantle Plumes: Some Phenomenology.” Journal of Geophysical Research 95, no. B5 (May 10): 6715–6736.
Sleep, Norman H. 1992. “Hotspot Volcanism and Mantle Plumes.” Annual Review of Earth and Planetary Science 20: 19–43.
Sleep, Norman H. 1997. “Lateral Flow and Ponding of Starting Plume Material.” Journal of Geophysical Research 102, no. B5 (10 May): 10001–10012.
Smith, Walter H. F., and David T. Sandwell. 1997. “Global Sea Floor Topography from Satellite Altimetry and Ship Depth Soundings.” Science 277, no. 5334 (26 September): 1956–1962.
Snelling, Andrew A. 2008. “Catastrophic Granite Formation: Rapid Melting of Source Rocks, and Rapid Magma Intrusion and Cooling.” Answers Research Journal 1 (February 6): 11–25.
Snelling, Andrew A., Martin Ernst, Esther Scheven, Joachim Scheven, Steven A. Austin, Kurt P. Wise, Paul Garner, Michael Garton, and David Tyler. 1996. “The Geological Record.” Creation Ex Nihilo Technical Journal 10, no. 3 (December): 333–334.
Snelling, Andrew A., and John Woodmorappe. 1998. “The Cooling of Thick Igneous Bodies on a Young Earth.” In Proceedings of the Fourth International Conference on Creationism, edited by R. E. Walsh, 527–545. Pittsburgh, Pennsylvania: Creation Science Fellowship.
Snelling, Andrew A. and John Woodmorappe. 2009. The Cooling of Thick Igneous Bodies on a Young Earth.” Answers in Depth 4 (August 5): 14–31. https://answersingenesis.org/geology/the-cooling-of-thick-igneous-bodies-on-a-young-earth/.
Sobolev, Alexander V., Evgeny V. Asafov, Andrey A. Gurenko, Nicholas T. Arndt, Valentina G. Batanova, Maxim V. Portnyagin, Dieter Garbe-Schönberg, and Stepan P. Krasheninnikov. 2016. “Komatiites Reveal a Hydrous Archaean Deep-Mantle Reservoir.” Nature 531, no. 7596 (30 March): 628–632.
Sobolev, Stephan V., and Michael Brown. 2019. “Surface Erosion Events Controlled the Evolution of Plate Tectonics on Earth.” Nature 570, no. 7759 (5 June): 52–57.
Sparks, R. S. J., C. Annen, J. D. Blundy, K. V. Cashman, A. C. Rust, and M. D. Jackson. 2019. “Formation and Dynamics of Magma Reservoirs.” Philosophical Transactions of the Royal Society A: Mathematical, Physical and Engineering Sciences 377, no. 2139 (25 February): 20180019. doi: 10.1098/rsta.2018.0019.
Stacey, Frank D., and Paul M. Davis. 2008. Physics of the Earth. 4th ed. Cambridge, United Kingdom: Cambridge University Press.
Stein, Carol A., and Seth Stein. 1992. “A Model for the Global Variation in Oceanic Depth and Heat Flow with Lithospheric Age.” Nature 359, no. 6391 (10 September): 123–129.
Stein, Claudia, Mariano Mertens, and Ulrich Hansen. 2020. “A Numerical Study of Thermal and Chemical Structures at the Core-Mantle Boundary.” Earth and Planetary Science Letters 548 (15 October): 11698. doi: 10.1016/j.epsl.2020.116498.
Stenberg, Don. 2012. “A New Magnetic Field Theory and Flood Model—Part 2.” Journal of Creation 26, no. 2 (August): 63–69.
Stern, Robert J. 2005. “Evidence from Ophiolites, Blueschists, and Ultrahigh-Pressure Metamorphic Terranes that the Modern Episode of Subduction Tectonics Began in Neoproterozoic Time.” Geology 33, no. 7 (July): 557–560.
Stern, R. J., M. I. Leybourne, and Tatsuki Sujimori. 2016. “Kimberlites and the Start of Plate Tectonics.” Geology 44, no. 10 (October 1): 799–802.
Su, Wei-Jia, Robert L. Woodward, and Adam M. Dziewonski. 1994. “Degree 12 Model of Shear Velocity Heterogeneity in the Mantle.” Journal of Geophysical Research: Solid Earth 99, no. B4 (10 April): 4945–4980.
Sun, Chenguang, and C. Johan Lissenberg. 2018a. “Formation of Fast-Spreading Lower Oceanic Crust as Revealed by a new Mg–REE Coupled Geospeedometer.” Earth and Planetary Science Letters 487 (1 April): 165–178.
Sun, Chenguang, and C. Johan Lissenberg. 2018b. “Caveats and Challenges in Geospeedometry: A Reply to Faak et al.’s Critique of the Mg–REE Coupled Geospeedometry.” Earth and Planetary Science Letters 502 (15 November): 287–290.
Sun, Daoyuan, Don Helmberger, Sidao Ni, and Dan Bower. 2009. “Direct Measures of Lateral Velocity Variation in the Deep Earth.” Journal of Geophysical Research: Solid Earth 114, no. B5 (May): 303. doi:10.1029/2008JB005873.
Tang, Ming, Kang Chen, and Roberta L. Rudnick. 2016. “Archean Upper Crust Transition from Mafic to Felsic Marks the Onset of Plate Tectonics.” Science 351, no. 6271 (22 January): 372–375.
Taylor, Brian. 2006. “The Single Largest Oceanic Plateau: Ontong Java–Manihiki–Hikurangi.” Earth and Planetary Science Letters 241, nos. 3–4 (31 January): 372–380.
Taylor, Stuart Ross. 2001. Solar System Evolution: A New Perspective. 2nd ed. Cambridge, United Kingdom: Cambridge University Press.
Tepley, Frank J. III, and Jon P. Davidson. 2003. “Mineral-Scale Sr-Isotope Constraints on Magma Evolution and Chamber Dynamics in the Rum Layered Intrusion, Scotland.” Contributions to Mineralogy and Petrology 145, no. 5 (20 June): 628–641.
Thiede, David S., and Paulo M. Vasconcelos. 2010. “Paraná Flood Basalts: Rapid Extrusion Hypothesis Confirmed by New 40Ar/39Ar Results.” Geology 38, no. 8 (August): 747–750.
Thordarson, T., and S. Self. 1998. “The Roza Member, Columbia River Basalt Group: A Gigantic Pahoehoe Lava Flow Field Formed by Endogenous Processes?” Journal of Geophysical Research Solid Earth 103, no. Bll (10 November): 27411–27445.
Thorne, Michael S., Nozomu Takeuchi, and Katsuhiko Shiomi. 2019. “Melting at the Edge of a Slab in the Deepest Mantle.” Geophysical Research Letters 46, no. 14 (28 July): 8000–8008.
To, Akiko, Barbara Romanowicz, Yann Capdeville, and Nozomu Takeuchi. 2005. “3D Effects of Sharp Boundaries at the Borders of the African and Pacific Superplumes: Observation and Modeling.” Earth and Planetary Science Letters 233, nos. 1–2 (30 April): 137–153.
Torsvik, Trond H., Mark A. Smethurst, Kevin Burke, and Bernhard Steinberger. 2006. “Large Igneous Provinces Generated from the Margins of the Large Low-Velocity Provinces in the Deep Mantle.” Geophysical Journal International 167, no. 3 (December): 1447–1460.
Torsvik, Trond H., Bernhard Steinberger, Robin M. Cocks, and Kevin Burke. 2008. “Longitude: Linking Earth’s Ancient Surface to its Deep Interior.” Earth and Planetary Science Letters 276, nos. 3–4 (15 December): 273–282.
Torsvik, Trond H., Rob van der Voo, Pavel V. Doubrovine, Kevin Burke, Bernhard Steinberger, Lewis D. Ashwal, Reidar G. Trønnes, Susan J. Webb, and Abigail L. Bull. 2014. “Deep Mantle Structure as a Reference Frame for Movements in and on the Earth.” Proceedings of the National Academy of Sciences 111, no. 24 (June 2): 8735–8740.
Turcotte, Donald L., and Gerald Schubert. 2002. Geodynamics. 2nd ed. Cambridge, United Kingdom: Cambridge University Press.
Turner, Simon, and Fidel Costa. 2007. “Measuring Timescales of Magmatic Evolution.” Elements 3, no. 4 (August): 267–272.
Tyler, Robert H. 2021. “On the Tidal History and Future of the Earth–Moon Orbital System.” Planetary Science Journal 2, no. 2 (April): 70. doi: 10.3847/PSJ/abe53f.
Valley, John W., Peter D. Kinny, Daniel J. Schulze, and Michael J. Spicuzza. 1998. “Zircon Megacrysts from Kimberlite: Oxygen Isotope Variability Among Mantle Melts.” Contributions in Mineralogy and Petrology 133, nos. 1–2 (1 October): 1–11.
Van Avendonk, Harm J. A., Joshua K. Davis, Jennifer L. Harding, and Lawrence A. Lawver. 2017. “Decrease in Oceanic Crustal Thickness Since the Breakup of Pangaea.” Nature Geoscience 10 (12 December): 58–61. doi: 10.1038/ngeo2849.
Van Hunen, Jeroen, and Jean-François Moyen. 2012. “Archaean Subduction: Fact or Fiction?” Annual Review of Earth and Planetary Sciences 40 (May): 195–219.
Vardiman, L., Steven A. Austin, John R. Baumgardner, Steven W. Boyd, Eugene F. Chaffin, Donald B. DeYoung, D. Russell Humphreys, and Andrew A. Snelling. 2005. “Summary of Evidence for a Young Earth from the RATE Project.” In Radioisotopes and the Age of the Earth: Results of a Young-Earth Creationist Research Initiative, edited by Larry Vardiman, Andrew A. Snelling, and Eugene F. Chaffin, 735–772. El Cajon, California: Institute for Creation Research and Chino Valley, Arizona: Creation Research Society.
Wan, Zhihuan, Laurence A. Coogan, and Dante Canil. 2008. “Experimental Calibration of Aluminum Partitioning Between Olivine and Spinel as a Geothermometer.” American Mineralogist 93, no. 7 (July 1): 1142–1147.
Webb, A. Alexander G., Thomas Müller, Jiawei Zuo, Peter J. Haproff, and Anthony Ramirez-Salazar. 2020. “A Non-Plate Tectonic Model for the Eoarchean Isua Supracrustal Belt.” Lithosphere 12, no. 1 (January 30): 166–179.
Weber, Gregor, Luca Caricchi, José L. Arce, and Axel K. Schmitt. 2020. “Determining the Current Size and State of Subvolcanic Magma Reservoirs.” Nature Communications 11 (5 November): 5477. doi: 10.1038/s41467-020-19084-2.
Wen, Lianxing, and Donald V. Helmberger. 1998. “Ultra-Low Velocity Zones Near the Core-Mantle Boundary from Broadband PKP Precursors.” Science 279, no. 5357 (13 March): 1701–1703.
White, J. D. L., S. E. Bryan, P.-S. Ross, S. Self, and T. Thordarson. 2009. “Physical Volcanology of Continental Large Igneous Provinces: Update and Review.” In Studies in Volcanology: The Legacy of George Walker, edited by T. Thordarson, S. Self, G. Larsen, S. K. Rowland, and Á. Höskuldsson, 291–321. IAVCEI Special Publications 2. The Geological Society, London.
White, R. S., T. A. Minshull, M. J. Bickle, and C. J. Robinson. 2001. “Melt Generation at Very Slow-Spreading Oceanic Ridges: Constraints from Geochemical and Geophysical Data.” Journal of Petrology 42, no. 6 (June): 1141–1196.
White, William M. 2010. “Oceanic Island Basalts and Mantle Plumes: The Geochemical Perspective.” Annual Review of Earth and Planetary Sciences 38, no. 1 (April): 133–160.
Wignall, P. B. 2001. “Large Igneous Provinces and Mass Extinctions.” Earth-Science Reviews 53, nos. 1–2 (March): 1–33.
Wignall, Paul. 2005. “The Link between Large Igneous Province Eruptions and Mass Extinctions.” Elements 1, no. 5 (December 1): 293–297.
Wikipedia. 2019. “Susanna Drury.” https://en.wikipedia.org/wiki/Susanna_Drury.
Williams, George E. 2000. “Geological Constraints on the Precambrian History of Earth’s Rotation and the Moon’s Orbit.” Reviews of Geophysics 38, no. 1 (February): 37–59.
Wilson, J. Tuzo. 1963. A possible origin of the Hawaiian Islands. Canadian Journal of Physics 41: 863–870.
Wilson, D. J., A. H. Robinson, R. W. Hobbs, C. Peirce, and M. J. Funnell. 2019. “Does Intermediate Spreading-Rate Oceanic Crust Result from Episodic Transition between Magmatic and Magma-Dominated, Faulting-Enhanced Spreading?—The Costa Rica Rift Example.” Geophysical Journal International 218, no. 3 (September): 1617–1641.
Wilson, J. Tuzo. 1965. “Convection Currents and Continental Drift: Evidence from Ocean Islands Suggesting Movement in the Earth.” Philosophical Transactions of the Royal Societyof London. Series A, Mathematical and Physical Sciences 258, no. 1088. A Symposium on Continental Drift (October 28): 145–167.
Windley, Brian F., Tim Kusky, and Ali Polat. 2021. “Onset of Plate Tectonics by the Eoarchean.” Precambrian Research 352 (January): 105980. doi: 10.1016/j.precamres.2020.105980.
Wolfram MathWorld. 2022. “Riemann Zeta Function.” https://mathworld.wolfram.com/RiemannZetaFunction.html.
Wood, Bernard J., Michael J. Walter, and Jonathan Wade. 2006. “Accretion of the Earth and Segregation of its Core.” Nature 441, no. 7095 (15 June): 825–833.
Woodmorappe, John, and Michael J. Oard. 2002. “Field Studies in the Columbia River Basalt, Northwest USA.” TJ 16, no. 1 (April): 103–110.
Worraker, W. J. 2016. “Quantifying the Flood Heat Problem.” Journal of Creation Theology and Science Series C: Earth Sciences 6: 4–5.
Worraker, William J. 2018. “Heat Problems Associated with Genesis Flood Models—Part 1: Introduction and Thermal Boundary Conditions.” Answers Research Journal 11 (July 11): 171–191. https://answersresearchjournal.org/heat-problems-flood-models-1/
Worraker, William J. 2019. “Heat Problems Associated With Genesis Flood Models—Part 2. Secondary Temperature Indicators.” Answers Research Journal 12 (September 18): 211–254. https://answersresearchjournal.org/heat-problems-flood-models-2/.
Worraker, William. J. 2020. “Heat Problems Associated with Genesis Flood Models—Part 3: Vapor Canopy Models.” Answers Research Journal 13 (August 5): 69–94.
Worraker, William J., and Richard Ward. 2018. “Modeling of Flood and Post-Flood Ocean Floor Cooling.” In Proceedings of the Eighth International Conference on Creationism, edited by J. H. Whitmore, 673–682. Pittsburgh, Pennsylvania: Creation Science Fellowship. doi: 10.15385/jpicc.2018.8.1.45.
Yao, Jinlong, Peter A. Cawood, Guochun Zhao, Yigui Han, Xiaoping Xia, Qian Liu, and Peng Wang. 2021. “Mariana-Type Ophiolites Constrain the Establishment of Modern Plate Tectonic Regime During Gondwana Assembly.” Nature Communications 12 (7 July): 4189. doi: 10.1038/ 38/s41467-021-24422-z.
Yuan, Kaiqing, and Barbara Romanowicz. 2017. “Seismic Evidence for Partial Melting at the Root of Major Hot Spot Plumes.” Science 357, no. 6349 (28 July): 393–397.
Zhu, Wei, Patrick Baud, Sergio Vinciguerra, and Teng-fong Wong. 2011. “Micromechanics of Brittle Faulting and Cataclastic Flow in Alban Hills Tuff.” Journal of Geophysical Research: Solid Earth 116, no. B6 (June): B06209. doi: 10.1029/2010JB008046.
Appendix 1:
The “Plates Versus Plumes” Controversy, and the Significance of Large Mantle Heterogeneities
The history and geological issues involved in the plates versus plumes controversy have been summarized by Anderson and Natland (2005), Foulger (2012), and, from a geodynamic perspective, by Hamilton (2019). These authors are all largely skeptical of the mantle plumes hypothesis: a more even-handed view of the history is sketched by White (2010), who is essentially a supporter of the hypothesis; see also Bryan and Ferrari (2013), Ernst, Buchan, and Campbell (2005). The issue has been characterized by Foulger (2016) as basically a “shallow-lithosphere versus deep-mantle controversy”, and more informatively by Anderson and Natland (2005, 120) thus:
Plume theory emphasized fluid dynamics, active upwellings, and high temperature. The other theories emphasized lithospheric rheology, architecture, stress, shallow return flow, and passive convection, with cooling plates and slabs the active elements.
The plate tectonics paradigm became generally accepted by the earth science community in the 1960s (Frazier 1969; Gramling 2021). Holmes (1931) had previously suggested that the earth’s mantle (in his terms “substratum”) was in large-scale convective motion leading to continental drift and deformation; however, the giant convection cells in his portrayal bear little resemblance to the mantle plumes postulated from the 1970s onwards. The concept of crustal plate motion over fixed hotspots was proposed to explain volcanic activity unconnected with plate margins, the prime example being Hawaii and its associated island chain (Wilson 1963). Initially there was no clearly-defined convection mechanism in view (Wilson 1963; 1965), but subsequently buoyant thermal plumes rising from the lowest part of the mantle were proposed not only to explain Hawaiian-type island volcanism but also to provide the driving force for plate motion (Morgan 1971; 1972); this latter function has since been discredited (White 2010). Such plumes were associated with the rapid, large-scale volcanism of continental flood basalts (Campbell 2001, 2005, 2007; Campbell and Griffiths 1990; Griffiths and Campbell 1990; Richards, Duncan, and Courtillot 1989) and later, more broadly, with Large Igneous Provinces (Bryan and Ferrari 2013; Ernst 2007; Ernst and Buchan 1997; Ernst, Buchan, and Campbell 2005). Plumes were envisaged as mushroom-type diapirs (a term more commonly associated with salt domes), with a broad cooling head and hot narrow tail, a picture based partly on laboratory experiments with heated glucose syrup (Griffiths and Campbell 1990). However, the relevance of such experiments (performed in a Newtonian fluid at atmospheric pressure and employing unrealistic initial and boundary conditions) is highly questionable. Thus, for example, pressure in the mantle varies considerably with depth, there is compositional and temperature heterogeneity over a range of scales, and mantle rheology is both non-Newtonian and highly uncertain (Korenaga 2010; 2018; Larsen and Yuen 1997); even recent, high-resolution numerical simulations used to demonstrate the possible role of a hot mantle plume in initiating subduction and thereby kick-starting plate tectonics on earth in the Archean have assumed, without justification, the existence of the proposed plumes (Gerya et al. 2015). Nevertheless, the mantle plume hypothesis was taken up and enthusiastically developed by some researchers (for example, Sleep 1990; 1992; 1997), though others have sought to apply it more judiciously (for example Courtillot et al. 2003). According to Anderson and Natland (2005) the analysis of helium (specifically 3He) flow upwards through the mantle by Kellogg and Wasserburg (1990) was the key development to boost interest in and acceptance of the mantle plumes paradigm. Textbooks have tended to adopt this paradigm without question (Stacey and Davis 2008; Turcotte and Schubert 2002).
Rather than pursue the history of the debate further here, we consider how it looks now. Direct detection by finite-frequency tomography of mantle plumes, some of deep origin, was claimed by Montelli et al. (2004; 2006) from observations of seismic wave speeds. Although these authors find numerous seismic features, their shapes are often poorly defined, especially deep in the mantle where resolution is relatively poor; thus there is significant probability of finding apparent but unreal associations with hotspots. One of the problems here is how to quantify margins of uncertainty (Marignier, Ferreira, and Kitching 2020). A common current view of mantle plumes, based on advanced whole-mantle seismic imaging techniques, is that relatively few broad plumes arise from near the core-mantle boundary at ULVZs (or Ultra-Low Velocity Zones; see Avants, Lay, and Garnero 2006; Thorne, Takeuchi, and Shiomi 2019; Wen and Helmberger 1998), reaching up to about 1,000 km from the surface where they may be deflected by a considerable distance horizontally (French and Romanowicz 2015; Yuan and Romanowicz 2017); fig. A1.1, based on fig. 4 in Yuan and Romanowicz (2017), shows this concept in sketch form; this is a rather different picture from that envisaged by the early proponents of mantle plumes (Morgan 1971; 1972). However, recent research (Davaille and Romanowicz 2020—see below) suggests that these broad plumes or superplumes may in fact be bundles of much smaller plumes.
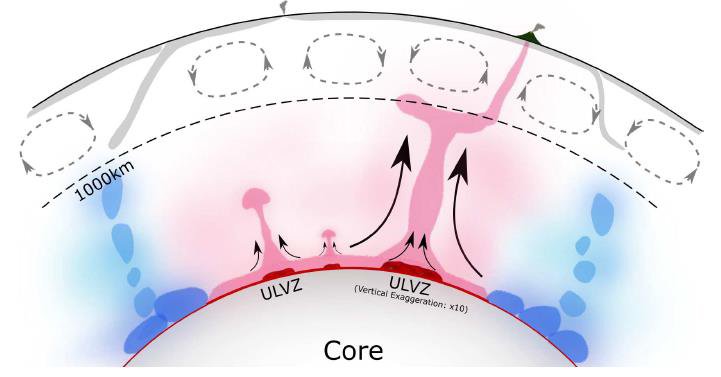
Fig. A1.1. Reproduction of fig. 4 in Yuan and Romanowicz (2017). Conceptual sketch of ULVZs (red) as topographic hills in a thin, dense, partially molten layer at the core-mantle boundary. The ULVZs are vertically exaggerated by a factor of 10 relative to the thickness of the mantle and the height of the plumes (pink). A large, axisymmetric ULVZ resides under the broad mantle plume, whereas smaller ones may exist beneath less fully developed upwellings. The thick dashed line indicates the depth of 1,000 km where many large plumes are deflected horizontally and some slabs (grey) stagnate. Sinking slab fragments are shown in blue. Arrows indicate the direction of flow.
Also very recently, investigators who do not fully subscribe to the mantle plumes paradigm have continued to produce coherent, defensible results from their own investigations without reference to plumes (Foulger, Schiffer, and Peace 2020; Foulger et al. 2020; Gernigon et al. 2020; Hole and Natland 2020). Other recent work on plate tectonics and mantle dynamics has a different emphasis. Thus some have approached this subject from the perspective of self-organization of a global convecting system in which mantle plumes play a relatively minor role (Coltice, Gérault, and Ulvrová 2017; Coltice et al. 2019). Crameri et al. (2019) focus on the life cycle of an oceanic plate as central in plate tectonics: here mantle plumes are part of the picture, but in the subsidiary role of “completing the circuit” in mantle convection, which is driven largely by surface cooling and subduction. Niu (2021) proposes lithosphere thickness as the key control on earth’s magmatic history, specifically the extent of mantle melting, the depth of melt extraction, and basalt compositions; he also asserts that this parameter controls whether mantle plumes surface or not and that LIPs serve as signs of thin or thinned lithosphere at the time of emplacement. Also, while accepting the occurrence of mantle plumes, Niu (2020) proposes that continental breakup is a straightforward consequence of plate tectonics without requiring mantle plumes, and concludes that superplumes do not exist. To summarize, today’s consensus picture is that mantle plumes do indeed exist, but are represented by much broader upwellings than envisaged in the 1970s, may be due to both thermal and chemical effects (French and Romanowicz 2015), and play only a passive role in mantle convection (Crameri et al. 2019); plate tectonics as observed today is driven primarily by the slab-pull force associated with subduction, which in turn is a consequence of heat loss through the ocean floors (Baumgardner 2013; Bédard 2018; Bercovici 2003; Bercovici, Ricard, and Richards 2000; Conrad and Lithgow-Bertelloni 2002; Crameri et al. 2019; Forsyth and Uyeda 1975; Turcotte and Schubert 2002)._
Mention should be made here of the largest known mantle heterogeneities, viz. the two Large Low Shear Wave Velocity Provinces (LLSVPs) on the core-mantle boundary. These are known by some earth scientists as Tuzo (beneath Africa) and Jason (beneath the Pacific Ocean) as noted in the main text; see fig. 1. These lie across the equator in roughly antipodal positions relative to each other. They were discovered seismically (Burke 2011; Garnero, Lay, and McNamara 2007; Torsvik et al. 2006; 2008), appear to be very long-lived, having existed at least since the beginning of the Cambrian (Burke 2011; Torsvik et al. 2014), and their edges, which are sharply defined in some places (Garnero, Lay, and McNamara 2007; Ni et al. 2002; To et al. 2005; Sun et al. 2009), are closely associated with LIPs and strong mantle plumes (Burke 2011; Torsvik et al. 2014). A recent popular description is given by Gorvett (2022). The ULVZs noted above are often associated with these much larger structures (Garnero, McNamara, and Shim 2016; Heron and Garnero 2019); fig. A1.2 illustrates the relationship of ULVZs and LLSVPs; note that this portrayal is observation-based rather than model-based. However, several aspects of these anomalous mantle regions remain controversial (Heron and Garnero 2019). Baumgardner (2003) has asserted that they are of relatively low density, also noting the existence of a ring of relatively high seismic wave velocity underlying the Pacific Rim (Su, Woodward, and Dziewonski 1994), which he interprets as signifying high density material resulting from subduction during the most active phase of the Flood. However, some seismologists have inferred relatively high density for the LLSVPs (Ishii and Tromp 1999; Lau et al. 2017), while others have found variable but relatively low density (Koelemeijer, Deuss, and Ritsema 2017). Niu (2018) argues strongly that LLSVPs have higher density than the surrounding lower mantle material, and that they were formed of subducted ocean crust accumulated over earth’s history. Some investigators, primarily interested in the origin of deep-mantle plumes, have been able to model LLSVPs as high-temperature, low-density regions in an isochemical mantle (Davies et al. 2012; Davies, Goes, and Lau 2015; Schuberth, Bunge, and Ritsema 2009). Others have treated them as chemical or thermochemical features (Davaille 1999; Garnero, McNamara, and Shim 2016; Koelemeijer, Deuss, and Ritsema 2017; McNamara and Zhong 2005; Stein, Mertens, and Hansen 2020), but it seems that this identification may be biased by long-age assumptions. The suggestion of Schubert et al. (2004) that LLSVPs consist of plume bundles rather than superplumes has recently been supported by Davaille and Romanowicz (2020), who argue in terms of fluid dynamics and higher-resolution seismic models than were previously available. A further possible complication in understanding the nature of LLSVPs lies in the suggestion that they may not be completely fixed on the CMB (Bono, Tarduno, and Bunge 2019). The long-term stability of LLSVPs and their association with LIPs is likely to be important for in-depth modelling of the Flood and its aftermath.
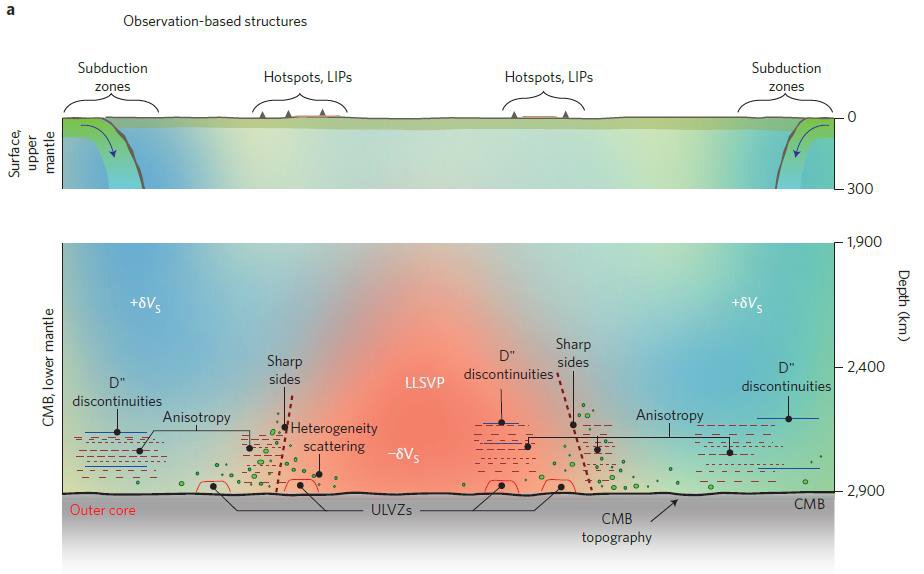
Fig. A1.2. Reproduction of fig. 2a in Garnero, McNamara, and Shim (2016). Summary in sketch form of LLSVP observations and interpretations. Surface features are shown in the upper panel and seismically determined lower-mantle phenomena in the lower panel. Note the spatial relationship between LLSVPs and ULVZs and their relative sizes depicted here in the lower panel.
In summary, the origin and significance of the LLSVPs are still not well established, whether viewed from a creationary or a conventional geological perspective, but remain important for a more complete description of earth structure and earth history. Further investigation from a creation science perspective, taking special account of the assumptions made in the mainstream literature, may prove fruitful.
Appendix 2:
The Onset of Plate Tectonics on Earth
The timing of plate tectonics onset is regarded by many authors as a vitally important topic, yet the answers given in the mainstream literature cover a wide range, from more than 4.0 Ga to the early Cambrian. Although papers addressing this subject generally cite a selection of relevant literature, for relatively wide-ranging reviews see Bédard (2018); Hawkesworth, Cawood, and Dhuime (2020); Korenaga (2013; 2018); Liu et al. (2019); Palin et al. (2020); Van Hunen and Moyen (2012); Windley, Kusky, and Polat (2021). The earliest estimates refer to Hadean times, for example 4.4 Ga (Ernst 2017), 4.37–4.20 Ga (Maruyama, Santosh, and Azuma 2018), >4 Ga (Hopkins, Harrison, and Manning 2008; 2010) and 4.02–3.75 Ga (Aarons et al. 2020), while the most recent are in the Neoproterozoic, notably 600 Ma (Hamilton 2019), ~1 Ga (Stern, Leybourne, and Sujimori 2016), and in some cases even extending into the Cambrian, for example ~1.0–0.5 Ga (Gerya 2019), ~525 Ma (Yao et al. 2021). The figures cited in connection with these papers should, however, be treated with caution, as they refer to a considerable variety of criteria for discerning the occurrence of plate tectonics. The wide diversity of answers in the literature addressing the question “When did plate tectonics begin on earth?” is attributed by Korenaga (2013, 118) to:
ambiguities in the interpretation of relevant geological observations as well as different weightings on different kinds of data.
Cawood et al. (2018, 19) emphasize the necessity of a global perspective and the integration of data of different kinds and from different regions:
We emphasize the need to avoid arguments built on observations from specific regions or data types, not least because of the difficulties in establishing the wider significance of localized datasets in highly heterogeneous material, such as the continental crust. Such information is important but it must be part of a multicomponent analysis to build geologically constrained and integrated global datasets.
See also Bédard (2018) and Palin et al. (2020). The diversity of proposed starting points for plate tectonics is thus probably due partly to different criteria, partly to the use of different datasets, to different ways of analyzing the data, to the limited scope of many investigations, to different research agendas, and to the backgrounds and even personal preferences of the investigators concerned. A key point is the assumed default state of the early earth, even though this is rarely made explicit. Thus, while most studies have implicitly assumed that earth’s earliest form of tectonic activity was unlike today’s, others have taken the opposite approach, for example, Kusky, Windley, and Polat (2018), who seek to place the burden of proof on those who assume a different form for the earliest earth tectonics; this leads Kusky, Windley, and Polat (2018) to conclude that plate tectonics has been operating at least since the formation of the earliest rocks, that is, from before the start of the Archean; see also Windley, Kusky, and Polat (2021). Critics of this approach may, however, see this conclusion as simply reflecting the investigators’ bias. Using very different starting assumptions Webb et al. (2020) present a non-plate tectonics model for the Isua Supracrustal belt in SW Greenland (conventionally dated between 3.8 and 3.7 Ga), which they note as “Earth’s only site older than 3.2 Ga that is exclusively interpreted via plate tectonic theory,” claiming that their model is simpler than existing plate-tectonic models of this area.
In another example of apparent bias Hamilton (2019, 47) identifies himself as contrarian, implying that he rejects the consensus view that since very early in geological history earth’s dynamics has been characterized by plate tectonics wherein lithosphere is carried right down into the lower mantle. His fig. 1 (based on fig. 3 in Stern, Leybourne, and Sujimori 2016) shows histograms for the past 3 Ga of earth history of the occurrence of kimberlites, ophiolites, blueschists, and glaucophane-bearing eclogites, ultra-high pressure (UHP) metamorphic rocks, lawsonite-bearing metamorphic rocks, and jadeitites, all generally regarded as distinctive plate tectonic and subduction indicators. However, in the caption to his own fig. 1 Hamilton (2019) says:
All classical ophiolites are included. Stern’s published diagram applied a dubious locally-expanded definition of “ophiolite” to add several very different Meso- and Neo-Proterozoic assemblages which I have removed here.
Hamilton (2019) is thus accusing Stern, Leybourne, and Sujimori (2016) of bias in their presentation of data, while looking biased himself in rejecting some of their data on grounds he leaves unexplained. Most of the diversity of estimates of the onset of plate tectonics, however, arises from the use of different criteria associated with different research interests together with the limited scope of individual studies. The following paragraphs illustrate this through short summaries of several papers concerned with the onset of plate tectonics.
Hopkins, Harrison, and Manning (2008) investigated the inclusion mineralogy of detrital Hadean igneous zircons from the Jack Hills of Western Australia, and from thermobarometric analyses of suitable inclusions determined that the zircons formed magmatically in conditions of about 700°C and 7 kbar. This result was taken to imply a near-surface heat flow of 75 mWm–2, ~3–5 times lower than typical estimates of Hadean global heat flow. The authors suggest that these Hadean zircons had crystallized from magmas formed largely in an underthrust environment similar to modern convergent margins. Analysis of a larger sample of Hadean zircons from the same area (Hopkins, Harrison, and Manning 2010) produced similar results. Greber et al. (2017) utilize the titanium isotopic ratio 49Ti/47Ti and its correlation with the SiO2 content of rocks to compare oceanic and continental crustal materials, the latter being much more felsic (silica-rich), and thus conclude that felsic crust was being produced from about 3.5 Ga; this was taken to signify plate tectonic activity. Aarons et al. (2020) employ the relationship between the titanium isotope ratio 49Ti/47Ti and the TiO2/SiO2 ratio in metamorphic rocks from the Acasta Gneiss Complex (in the NW Territories of Canada), which spans the Hadean/Eoarchean transition, claiming that their data documents a shift between 4.02 and 3.75 Ga from tholeiitic- to calc-alkaline-style magmatism, the latter being associated with subduction and hence the start of plate tectonics. Kirkland et al. (2021), whose primary interest is in the growth of continental crust, a process coupled with plate tectonics and magmatic fractionation, studied detrital zircons from modern stream sediment samples from West Greenland, dated (by the U-Pb system) in the range 3.9–1.8 Ga. They used Hf isotopic data combined with global zircon Hf data to deduce a planetary shift towards more juvenile Hf values in the interval 3.2–3.0 Ga. This shift was called a crustal rejuvenation event coinciding with peak mantle potential temperatures implying greater degrees of mantle melting and injection of hot mafic-ultramafic magmas into older Hadean-to-Eoarchean felsic crust. From a study of trace and rare earth element geochemical data along with Hf and O isotope data of detrital zircons from the Green Sandstone Bed, Barberton Greenstone Belt (South Africa), Drabon et al. (2022) concluded that long-lived protocrust formed in the Hadean and was destabilized globally from no later than ~3.8 Ga, perhaps by the onset of at least sluggish mobile-lid tectonics.
El Dien et al. (2020) claim to have documented the onset of mantle re-enrichment through analysis of Nd isotope systematics and key ratios of highly incompatible elements compared to lithophile elements in Archean to early Proterozoic mantle-derived melts, viz. basalts and komatiites. They infer a rapid change in mantle chemistry around 3.2 Ga, interpreted as a fundamental change in earth geodynamics signifying the onset of global subduction processes associated with plate tectonics. Dhuime et al. (2012), also concerned with the growth of continental crust, studied the U-Pb and Lu-Hf systematics and δ18O values of detrital and inherited zircons from several parts of the world (Australia, Eurasia, North and South America). The point of considering oxygen isotopes was to distinguish between new and reworked crust, given that zircons crystallizing from mantle-derived magmas exhibit a narrow δ18O range, typically 5.3±0.6‰ in terms of a 2σ uncertainty margin (Valley et al. 1998). Dhuime et al. (2012) deduced that there was a significant reduction in the rate of new crust generation at ~3 Ga, which they interpreted as reflecting the onset of subduction-driven plate tectonics and discrete subduction zones.
From an extensive geochemical database Condie, Aster, and van Hunen (2016) used incompatible element distributions to characterize the magma generation temperatures (denoted Tg) of greenstone basalts and komatiites through time. Four types of mantle were investigated in this way: depleted, hydrated, enriched, and komatiite-producing mantle. While komatiite-producing mantle was consistently the hottest throughout (Tg falling from ~1700°C at 4 Ga to ~1600°C today), depleted and enriched mantle gave similar Tg values (just above 1500°C) until about 2.5 Ga, after which they diverged progressively, depleted (or ambient) mantle Tg values falling to 1350°C by the present day while the enriched mantle Tg, which the authors associated with plumes, only fell to about 1500°C. These thermal histories were interpreted as showing growing mantle depletion, mantle cooling due to declining radiogenic heating, and the propagation of plate tectonics from 3 Ga onwards. Condie (2018) notes ten specific geological and geochemical changes (including the appearance of ophiolites and occurrence of global LIP events) recorded between 3 and 2 Ga, and links these to cooling of the mantle, with corresponding changes in convective style and lithospheric strength; he suggests that these may record the gradual onset and propagation of plate tectonics—in conventional terms over a billion-year interval.
Some authors investigate data from sedimentary rocks, interpreted as older detrital material carrying information from its source. Thus Tang, Chen, and Rudnick (2016) used Ni/Co and Cr/Zn ratios in Archean terrigenous sedimentary rocks and Archean igneous/metaigneous rocks to track the bulk MgO composition of the Archean upper continental crust. They inferred that this crust had evolved from a highly mafic bulk composition before 3.0 Ga to a felsic bulk composition by 2.5 Ga, a change attended by a fivefold increase in the mass of the upper continental crust due to addition of granitic rocks, whence they deduced the onset of global plate tectonics at ~3.0 Ga. Sobolev and Brown (2019) consider and model the lubricating influence of sediment on subduction in a plate tectonic paradigm and then exhibit data from a range of proxies (T/P values of metamorphic rocks, number and lifespan of passive margins, geographical length of orogens, Sr isotope ratios in seawater, Hf and oxygen isotope ratios in detrital zircons, and number of LIPs) taken to indicate plate tectonic activity through time. Their overall conclusion was that before 3 Ga the tectonic regime was squishy lid (or of plume- or impact-induced retreating subduction type) in character, followed by evolution between 3–2 Ga into a global plate tectonic regime enabled by the rise of the continents and increased surface erosion. Pastor-Galán et al. (2018) link the onset of plate tectonics to the supercontinent cycle. They do not offer a definite conclusion, but point out the implications of both a very early date (that is, in the early Archean, in which case supercontinents must have formed independently of global mantle dynamics) and a late date (that is, ~750 Ma, which would invalidate the usual understanding of the supercontinent cycle, while implying that most of earth’s continental crust did not form by subduction). Stern (2005) also links subduction-driven plate tectonics with the Wilson supercontinent cycle: he considers the earliest evidence of ophiolites, blueschists, and UHP (ultra-high pressure) terranes, citing ages of 1.03 Ga for the oldest ophiolites, 800 Ma for the oldest blueschists, stating that the latter are older than the oldest UHP terranes. He claims that this is the expected order for the subduction-driven Wilson-supercontinent tectonic cycle and specifically from the sequence beginning with Rodinia break-up and culminating in the formation of a late Neoproterozoic supercontinent: hence his conclusion that the modern episode of subduction or plate tectonics began in Neoproterozoic time, i.e. between about 1 Ga and the beginning of the Cambrian (~0.54 Ga).
The above examples illustrate the different approaches and answers in the literature to the question of when plate tectonics began on earth; numerous other references could be cited. There is clearly no consensus. From a creation science perspective, which necessarily involves much shorter timescales, the most natural starting-point for vigorous tectonic activity, at least in CPT-based Flood models, would be the onset of the Flood (Genesis 7:11). However, this does not preclude earlier tectonic activity, doubtless supernaturally-controlled and extremely rapid in days 2 and 3 of Creation Week (Genesis 1:6-10), and much slower from then until the Flood began. Within this biblical time frame there are various modelling options for creation scientists, but these are beyond the scope of this paper.
Appendix 3:
Blackbody Radiation in N Spatial Dimensions
The dependence of blackbody radiation on the temperature of the radiating surface or hypersurface takes the form of a power law. On thermodynamic grounds the power takes the integer value N+1, where N is the number of spatial dimensions (Alnes, Ravndal, and Wehus 2007), and statistical mechanics gives the actual value of the necessary constants in the relationship. Thus Cardoso and Castro (2005) derive the following generalized Stefan-Boltzmann law for the total rate of blackbody radiation emitted by an (N-1)-dimensional surface (or hypersurface) in an N-dimensional space:
where RT is the blackbody radiance at absolute temperature T and σN is the Stefan-Boltzmann constant in N dimensions, given by
where c is the canonical velocity of light, kB is the Stefan-Boltzmann constant, h Planck’s constant, Γ the gamma function defined by Γ(1) = 1 and Γ(z+1) = z Γ(z), and ζ the Riemann zeta function defined by
where the real part of z must be >1. For real values of z and z>1, ζ(z) may also be defined by
We evaluate (A3.2) for N = 3, corresponding to 3D space, and N = 4, the case of special interest in connection with the Flood Heat problem.
For N = 3, note that
(Wolfram MathWorld 2022). Substituting these results in (A3.2) and simplifying then gives
For N = 4, the case of radiation from a 3D volume into a 4D hyperspace, note that Г(2) = Г(1) = 1 and ζ(5) ≈ 1.036927755 (Wolfram MathWorld 2022); note that ζ(5) is an irrational number. Substituting in (A3.2) and simplifying then gives
Substituting the known values of the parameters in the right-hand side of (A3.5) then gives σ3 = 5.67037442 × 10-8 Wm-2 K-4, the recognized value for 3D space. The same procedure applied to (A3.6) gives σ4 = 3.02064572 × 10-5 Wm-3K-5. Note that σ4 is defined in terms of unit volume of the emitter, corresponding to volume-based emission, as distinct from the familiar surface-based emission in 3D space implicit in the units of σ3.
To estimate the time for ocean floor magma of density ρ and specific heat Cp (ignoring latent heat effects) subject to thermal radiation in 4D to cool from temperature T0 to T1 we start with the heat balance equation
which integrates to
Alternatively, in terms of total heat deposition Q per unit volume, the cooling time may be written
where the expression ρCp(T0–T1) has been replaced by Q to avoid the need to estimate values for ρ and CP. Thus for ε = 1.0, average heat deposition 3.9 × 109 J/m3
through the depth of the oceanic lithosphere (Furlong and Chapman 2013) and initial and final average temperatures of 1500 K (as suggested by Humphreys 2018) and 600 K, cooling is complete within 0.4 seconds; if the emissivity for the case of a high speed of light in the fourth spatial dimension is ε = 1.652 × 10-6 (see above), this cooling time is increased to about 1.9 days, still extremely short in comparison with timescales associated with natural heat transfer processes.
Appendix 4:
Introduction to Diffusion Chronometry
Diffusion chronometry, sometimes called geospeedometry, is an important method of estimating cooling rates and cooling times (Chakraborty 2006; 2008; Costa, Dohmen, and Chakraborty 2008; Costa, Shea, and Ubide 2020; Costa 2021); the work of Dodson (1973; 1976) is foundational in this field. Diffusion processes are ubiquitous in non-equilibrium conditions, and are especially suited to measuring durations independently of both absolute geological age and nuclear decay rates. In the context of magma cooling and solidification, the key processes are solid- and liquid-state diffusion of elements and/or isotopes, often from one mineral to another. The timescale or duration of a particular process depends on the length scale involved and the relevant diffusion coefficient, which in turn depends on the diffusing species, the minerals and fluids involved, oxygen fugacity, and the temperature and pressure. Useful time-related information can only be obtained when the process has significantly advanced from its starting point but is not yet approaching equilibrium, when no further change is detectable; fig. A4.1 (based on fig. 1 in Chakraborty 2008) illustrates the general principles. Since diffusion rates of different elements in the various minerals are spread over many orders of magnitude, it is almost always possible to find an element or isotope that fits the window of opportunity (fig. A4.1) for any given process. In this connection, the rapidly improving capability of measuring trace element and isotopic concentration gradients with increasing spatial resolution is vital. However in practice diffusion chronometry is complex to use, demanding very precise measurements and extreme care in data interpretation (for example, Shea, Lynn, and Garcia 2015).
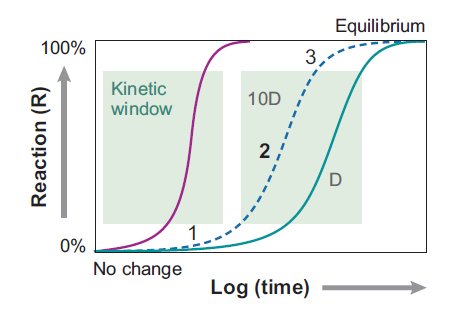
Fig. A4.1. Reproduction of fig. 1 in Chakraborty (2008). A generalized diagram to illustrate the evolution of diffusion processes (shown here as percent R) and their utility as clocks. (1) (R ~ 0%), (2) R(t), and (3) (R = 100%). To clock any geological process, it is necessary to identify physicochemical processes (radioactive decay, diffusion, deformation, etc.) with characteristic timescales that coincide with stage 2 (the “kinetic window,” shown here in light green). The incomplete transformation in stage 2 also needs to be frozen in the rock record so that it may be retrieved quantitatively at a later time. Diffusion in solid silicates fulfils both these requirements. Also shown are (a) the effect of uncertainties in our knowledge of rates of transformation (here, diffusion coefficients). An error/uncertainty in this quantity of an order of magnitude (rate = D versus 10 D) translates to an error in the determined duration of a process of the same magnitude (for a given observed degree of transformation, R). This highlights the necessity of knowing kinetic rates accurately. (b) The versatility of diffusion processes. It is usually possible to find diffusion coefficients of some element in some mineral that lies in stage 2 of any geological process of interest. For a process that is too fast to be clocked (that is, lying to the left of the kinetic window) by the diffusion of elements marked by the green lines (solid or dashed), a different diffusion process (purple line) can be found whose kinetic window lies in the right region. Examples may be diffusion of different elements in a single mineral such as plagioclase (Li: fast, Mg: intermediate, NaSi-CaAl: slow).
An example of the use of geospeedometry is the work by Coogan, Jenkin, and Wilson (2002) in assessing the cooling rate of newly formed lower oceanic crust by considering the closure temperature (the temperature at which diffusion effectively stops) of down-temperature diffusive exchange of Ca from olivine to clinopyroxene in gabbros from the Cretaceous Samail Ophiolite in Oman, a large slab of oceanic crust overthrust onto continental crust. However, absolute cooling rates in that case could not be determined because of uncertainty in the diffusion coefficient for Ca in olivine. Following an update of this parameter, the same authors (Coogan, Jenkin, and Wilson 2007) combined two methods, the down-temperature diffusive exchange of Ca between olivine and clinopyroxene and the down-temperature diffusive exchange of Mg and Fe between olivine and spinel, to assess the importance of magma advection and hydrothermal activity in comparison with heat conduction in the vicinity of both fast- and slow-spreading ridges. In another example, minimum cooling timescales for plagioclase crystals in Unit 10 and across the Unit 9/10 interface of the Rum layered intrusion in northwest Scotland were estimated on the basis of Sr isotope heterogeneities (variations in 87Sr/86Sr) across zones within single crystals to be ‘a few thousand years’ (Tepley and Davidson 2003); the length scale in this case was 1–2 mm. More recently, Hepworth et al. (2020) investigated Sr isotope heterogeneities between plagioclase crystals, between clinopyroxene and plagioclase, and within plagioclase crystals through centimeter-thick precious-metal-enriched layers from Unit 10 of the same layered intrusion on Rum. They found heterogeneities over 10–100 micron length scales, which implies that the melts from which the precious-metal-rich layers formed cooled at rates faster than 1°C per year; cooling to diffusive closure was thus complete within tens to hundreds of years (see fig. A4.2). It seems that this tightened constraint on maximum cooling times is due at least in part to the improved spatial resolution (10–100 microns versus 1–2 mm) available to Hepworth et al. (2020). Since for any chosen diffusion process the upper limit on cooling times depends critically on the smallest spatial scale which can be resolved, many published cooling times obtained by diffusion chronometry are likely to be too long. Estimated cooling times are thus likely to become shorter as the technology advances.
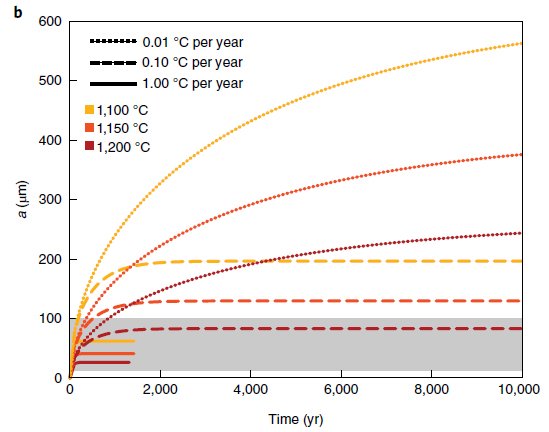
Fig. A4.2. Reproduction of fig. 4b in Hepworth et al. (2020) showing a plot of calculated length scale of Sr diffusion (a) versus time (yr) for anorthite. Sr isotope variations exist over length scales of 10–100 microns (grey shaded field along base of plot), so only curves contained completely within the field can be reconciled with the observations of Hepworth et al. (2020).
In recent years the concern for soundly-based methods of predicting volcanic eruptions and their potential impact on human safety and the terrestrial environment has prompted considerable effort in determining the timescales involved in the evolution of crustal magma chambers and their resulting eruption characteristics (for example, Biggs and Annen 2019; Cooper 2019; Edmonds et al. 2019; Jackson, Blundy, and Sparks 2018; Karakas et al. 2017; Rubin et al. 2017; Sparks et al. 2019; Weber et al. 2020). Diffusion chronometry is now commonly used in this context to assess storage and residence times of magma associated with active or potentially active volcanoes (e.g. Cashman, Sparks, and Blundy 2017; Cooper 2019; Morgan et al. 2004; Turner and Costa 2007). The development of this technique has occurred in parallel with a changing perspective among volcanologists on the real nature of magmatic systems, which are now recognized as considerably more complex than previously thought. Typically they are vertically and/or laterally extensive and are dominated by crystal mush, with only transient accumulations of crystal-poor melt. Combined with the high inferred crystallinity of most sub-volcanic systems, this recognition has compelled volcanologists to rethink the processes responsible for both magma evolution and volcanic eruptions, notably those related to redistribution of melt and crystals within magma storage regions (Cashman, Sparks, and Blundy 2017; Cashman and Edmonds 2019; Lipman and Bachmann 2015; Sparks et al. 2019). A particular problem in modelling igneous processes is that magma chambers are very difficult to form and maintain over conventional geological timescales because high rates of hot magma flowing into the upper crust are needed, leading to rapid crustal thickening and surface uplift, neither of which is observed. This has also prompted a rethinking of the structure and dynamics of magma chambers (Glazner 2021); in a creation science framework this issue may be an indication of shorter than conventional timescales associated with volcanism and hence perhaps calling for further investigation.
As an example of this use of diffusion chronometry, Gordeychik et al. (2018) examined Fo (forsterite) and Ni zoning patterns in high-Mg olivine crystals in tuff from Shiveluch volcano, Kamchatka and found that magma ascent during eruptions was extremely fast, taking only 1–10 days. Zircons from an eruption within the Taupo Volcanic Zone, New Zealand were examined by Rubin et al. (2017) to assess their thermal history using a combination of 238U-230Th dating of zones within single crystals and Li diffusion chronometry applied to the same zones. The results were interpreted as showing long periods (~103–105 years) of cold storage at near-solidus temperatures (~600–700°C) punctuated by rapid heating and cooling events lasting only years to centuries; these results caused some controversy (Costa, Shea, and Ubide 2020).
Note the following summary comments in the review of diffusion chronometry by Costa, Shea, and Ubide (2020, 12):
Finally, there is a large discrepancy between the timescales recorded by diffusion chronometry and U-Th disequilibria dating in magmatic zircons from large silicic eruptions. Although timescales from diffusion chronometry and U-Th disequilibria can be obtained in a single zircon, it is puzzling why diffusion chronometry records shorter timescales than U-Th disequilibria. The thermal history of the magma reservoir is one of the key factors that might help to explain this discrepancy.
These comments naturally invite further investigation of the use of zircons and other crystals for magmatic timescale assessment, whether from a biblical or uniformitarian perspective of earth history. For creation scientists the possibility of accelerated nuclear decay, implying that conventional U-Th timescales may generally be too long, further suggests that diffusion chronometry is a potentially fruitful field of investigation for the future.
Glossary
Adiabat. A line in a phase diagram (often a pressure-temperature diagram) corresponding to processes in which no heat transfer to or from the environment takes place.
Aphyric. Refers to the texture of a fine-grained or aphanitic igneous rock that lacks phenocrysts. Also, said of a rock exhibiting such texture.
Cation. A positively charged ion. Most metal ions in solution exist as cations, for example, Li+, Mg++, Ca++.
Entablature. The term used to describe zones or tiers of irregular jointing in basaltic lava flows. It is thought to form when water from rivers dammed by the lava inundates the lava flow surface, and during lava-meltwater interaction in subglacial settings (Forbes, Blake, and Tuffen 2014).
Fugacity. Variable used in chemical thermodynamics. The fugacity of a real gas is an effective partial pressure which replaces the mechanical partial pressure in an accurate computation of chemical equilibrium conditions. It is equal to the pressure of an ideal gas which has the same temperature and molar Gibbs free energy as the real gas. Oxygen fugacity (fO2) is a key variable in petrology, as it indicates the potential for iron to exist in a more oxidized or a more reduced state. At very low values (for example, as in the earth’s core) iron exists as a metal (Fe0). At higher values of fO2 and in silicate-bearing systems, iron occurs as a divalent cation and is mostly incorporated into silicates (for example, as fayalite, Fe2SiO4). At higher values still iron occurs in both divalent (ferrous) and trivalent (ferric) states, and is mostly incorporated into magnetite (Fe3O4), and at the highest values it exists as haematite (Fe2O3); see Frost (1991).
Ignimbrite. Rock composed of pumice fragments, formed by consolidated material from a pyroclastic flow.
Isopleth. A line in a phase diagram corresponding to a given value of an important parameter, for example, a given weight percentage of MgO in a dry peridotite magma. See, for example, fig. 2 in Hole and Natland (2020).
Liquidus and Solidus. The liquidus is the line in a phase diagram separating the field of all liquid from that of liquid plus crystals. The solidus is the line separating the field of all solid from that of liquid plus crystals. For examples see fig. 2 in Hole and Natland (2020).
Newtonian and non-Newtonian flows. A Newtonian flow is characterized by a linear relationship between the local stress and rate-of-strain tensors. In the simplest case, a one-dimensional shear flow, the ratio of the shear stress to the velocity gradient is termed the dynamic viscosity. The flows of most common fluids (for example, air, water, cooking oil, petrol) can be treated as Newtonian. However a wide range of flows of other substances cannot accurately be described in terms of a viscosity: these are non-Newtonian flows. The main classes of non-Newtonian behavior are (i) inelastic, or Generalized Newtonian flow, in which viscosity is treated as a function of the local shear rate; (ii) viscoelastic flow, in which part of the stress field can be related to elastic or solid-like behavior. This can also be thought of in terms of the fluid possessing a memory of its flow history; (iii) time-dependent behavior, in which the apparent viscosity corresponding to a given shear rate changes with time; such behavior is associated with changing internal structure in the fluid. If the apparent viscosity decreases with time the flow is called thixotropic; the converse (increase of apparent viscosity with time) is rheopectic behavior.
Ophiolite. An ophiolite is a section of oceanic crust and the underlying upper mantle that has been uplifted and exposed above sea level and often emplaced or obducted onto continental crustal rocks.
Pāhoehoe. A term describing the form of a gently-flowing basaltic lava stream, characterized by smooth, gently undulating, or broadly hummocky surfaces. The liquid lava flowing beneath a thin, still-plastic crust drags and wrinkles it into tapestry-like folds and rolls resembling twisted rope.
Phenocryst. A relatively large crystal embedded in a finer-grained or glassy igneous rock, giving the whole a porphyritic texture. Most common phenocryst minerals: feldspar, quartz, biotite, hornblende, pyroxene and olivine.
Redox. A redox reaction takes place between an oxidizing substance and a reducing substance. Oxidation is the loss of electrons or an increase in the oxidation state of a chemical or atoms within it, for example, by the addition of oxygen. Reduction is the gain of electrons or a decrease in the oxidation state of a chemical or atoms within it, for example, by the removal of oxygen. Examples and further details are given here: https://www.britannica.com/science/oxidation-reduction-reaction/Historical-origins-of-the-redox-concept.
Spinifex. A rock texture formed by skeletal, platy, or acicular crystals of olivine, orthopyroxene, or clinopyroxene, or their pseudomorphs in ultramafic and mafic lavas. It results from rapid crystallization of highly magnesian liquid in the thermal gradient at the margin of a flow or sill. Often seen in komatiites.
Types of volcanic eruption: Britannica (2021) lists six distinct types of volcanic eruption: (1) Icelandic, characterized by effusions of molten basaltic lava flowing from long, parallel fissures. Such outpourings often build lava plateaus; (2) Hawaiian (similar to Icelandic), in which fluid lava flows from a volcano’s summit and radial fissures to form shield volcanoes, which are quite large and have gentle slopes; (3) Strombolian eruptions involve moderate bursts of expanding gases that eject clots of incandescent lava in cyclical or nearly continuous small eruptions; (4) Vulcanian, which generally involve moderate explosions of gas laden with volcanic ash. This mixture forms rapidly ascending dark, turbulent eruption clouds which often assume contorted shapes; (5) Pelean eruptions undergo explosive outbursts which generate pyroclastic flows, dense mixtures of hot volcanic fragments and gas. The resulting fluidized slurries are more dense than air but have low viscosity and pour down valleys and slopes at great speeds. As a result, they are extremely destructive; (6) Plinian, in which gases boiling out of gas-rich magma generate enormous, almost continuous jetting blasts that core out the magma conduit and rip it apart. Plinian eruption clouds can reach the stratosphere. Sometimes they are produced continuously for several hours, and are often accompanied by lightning strikes due to a build-up of static electricity.
Urey ratio. The ratio of the earth’s internal heat production (due to core differentiation, radionuclide decay etc.) to surface heat flux. Korenaga (2008a; 2008b) distinguishes between the bulk earth Urey ratio, which refers to the whole earth and is preferred by geochemists, and the convective Urey ratio, the ratio of internal heat generation in the mantle to the mantle heat flux, which is more often used by geophysicists.
Rocks and Minerals
Only a few descriptions are given here. For formal definitions readers are referred to Le Bas and Streckeisen (1991); see also Le Maitre et al. (2002).
Eclogite. Description from https://www.mindat.org/min-48628.html: “A high P/T metamorphic rock composed of more than 75% vol. garnet (often pyrope or Mg-rich almandine) and omphacite (Na-Ca-Al-Mg clinopyroxene). Both must be present and neither component more than 75% vol. Eclogite never contains plagioclase. Rutile, kyanite, and quartz are typically present.”
Komatiites. Ultramafic rocks with high MgO content, and generally seen as representing primary melts generated by high degrees of partial melting and signifying high crystallization temperatures (Echeverria 1980; Nisbet et al. 1993). With the exception of Gorgona Island they are found mainly in Precambrian rocks. Nisbet et al. (1993) deduced maximum eruption temperatures in the Archaean of 1580°C, corresponding to mantle potential temperatures up to 1900°C (about 300°C higher than the contemporary average). Herzberg (1992) also found very high formation temperatures, but concluded that the melt fraction was not always very high. These temperature estimates are based on the assumption of dry magma (Arndt et al. 1998), but if water was present at sufficient concentration (of order 1 wt% or more), they would be significantly reduced. Grove and Parman (2004) suggested the possibility that komatiites were produced by hydrous melting at shallow mantle depths in a subduction environment, implying an average mantle temperature at 2.5 Ga (that is, in the Archaean) no more than ~100°C higher than today. Sobolev et al. (2016) also demonstrate how komatiite crystallization temperatures are reduced by the presence of water at concentrations of order 1 wt%.
Olivine. A group of silicate minerals found in mafic and ultramafic rocks. Basic formula: (Mg,Fe)2SiO4, where Mg and Fe proportions are freely variable. Series end-members are Mg2SiO4 (forsterite) and Fe2SiO4 (fayalite). The Mg and Fe can occasionally be substituted by Ca, Mn or Ni. Crystal system is orthorhombic.
Peridotites. Ultramafic igneous rocks (SiO2 content <45% by weight) containing at least 40% olivine, accompanied by varying proportions of pyroxenes (clinopyroxene and orthopyroxene), plus typically smaller proportions of chromite, plagioclase and amphibole (or hornblende).
Picrite. Picrite basalt or picrobasalt is a variety of high-magnesium olivine basalt that is very rich in the mineral olivine. It is dark with yellow-green olivine phenocrysts (20–50%) and black to dark brown pyroxene, mostly augite. The olivine-rich picrite basalts that occur with the more common tholeiitic basalts of Kīlauea and other volcanoes of the Hawaiian Islands are the result of accumulation of olivine crystals either in a portion of the magma chamber or in a caldera lava lake. The compositions of these rocks are well represented by mixes of olivine and more typical tholeiitic basalt. The name picrite can also be applied to an olivine-rich alkali basalt: such picrite consists largely of phenocrysts of olivine and titanium-rich augite pyroxene with minor plagioclase set in a groundmass of augite and more sodic plagioclase and perhaps analcite and biotite. Picrites and komatiites are somewhat similar chemically, but differ in that komatiite lavas are products of more magnesium-rich melts, and good examples exhibit the spinifex texture. In contrast, picrites are magnesium-rich because crystals of olivine have accumulated in more normal melts by magmatic processes. Komatiites are largely restricted to the Archean. https://en.wikipedia.org/wiki/Picrite_basalt.
Plagioclase. A series of tectosilicate (framework silicate) minerals within the feldspar group. Rather than referring to a particular mineral with a specific chemical composition, plagioclase is a continuous solid solution series, more properly known as the plagioclase feldspar series. The series ranges from albite to anorthite end members (with respective compositions NaAlSi3O8 to CaAl2Si2O8), where sodium and calcium atoms can substitute for each other in the mineral’s crystal lattice structure. Plagioclase in hand samples is often identified by its polysynthetic crystal twinning or record-groove effect. Plagioclase is a major constituent mineral in the earth’s crust, and is consequently an important diagnostic tool in petrology for identifying the composition, origin and evolution of igneous rocks. https://en.wikipedia.org/wiki/Plagioclase.
Pyroxenes. A group of dark inosilicate minerals formed at high temperature and/or pressure. General chemical formula has the form XYZ2O6, where X is Ca, Na, Fe++, Mg, Zn, Mn, or Li (or some combination), Y is Mg, Fe+++, Fe++, Cr, Al, Co, Mn, Sc, Ti, or Vn (or some combination) and Z is Si, Al, or a combination of both. A wide variety of cation substitutions can occur in the X and Y positions. Clinopyroxenes are pyroxenes which crystallize in the monoclinic system, while orthopyroxenes follow the orthorhombic system. Some relatively well-known examples of clinopyroxenes are augite [(Ca,Na)(Mg,Fe,Al,Ti)(Si,Al)2O6], diopside [CaMgSi2O6], jadeite [Na(Al,Fe+++)Si2O6] and spodumene [LiAl(SiO3)2], and examples of orthopyroxenes are hypersthene [(Mg,Fe)SiO3] and donpeacorite [(Mn++,Mg)Mg(SiO3)2].