The views expressed in this paper are those of the writer(s) and are not necessarily those of the ARJ Editor or Answers in Genesis.
Abstract
We report sedimentary structures that in all ways resemble parabolic recumbent folds (PRFs) in the cross-bedded portions of the Schnebly Hill Formation, the Coconino Sandstone and the Toroweap Formation (Arizona, USA). Field evidence suggests they are penecontemporaneous and intraformational. Intraformational refers to deformation that occurs between undeformed beds. Recumbent cross-bed sets occur over a wide area (>375 km2 [144 mi2]) at many different locations and horizons in the Sedona area, especially within the Coconino Sandstone. Deformation resulting from slumping dunes (dry or damp) is ruled out because of the nature of the deformation along cross-bed dip, the size and length of the deformation along horizontal bedding planes (sometimes up to 170 m [557 ft] along dip) and the lack of small faults usually concurrent with such slumping known from modern dunes. Neither do the folds resemble deformation that has been caused by post-depositional groundwater movement or seismic activity which often produces convolute bedding. We do report some seismic features in the Schnebly Hill Formation, but these features have distinct characteristics that distinguish them from PRFs.
Although the exact mechanism of PRF formation is still debated, it is generally agreed that strong water currents combined with liquefaction play major roles in overturning the top of a cross-bed set during the deposition of the cross-bed. Rare planar-beds, directly associated with the PRFs in the Coconino, suggest that the needed liquefaction may have occurred from changes in flow regime. Some workers have already suggested that parts of the Schnebly Hill and Coconino were deposited by marine sand waves on a shallow continental shelf; a hypothesis that is considerably strengthened in light of these new data along with additional petrographic data that we have collected.
Keywords: Coconino Sandstone, Schnebly Hill Formation, Toroweap Formation, parabolic recumbent folds, cross-bedded sandstones, soft-sediment deformation, liquefaction, primary current lineation, cross-bed dips
Introduction and Background
The Coconino Sandstone is a prominent Permian cross-bedded sandstone that outcrops over much of northern and central Arizona, including the Grand Canyon. It is characterized by fine-grained sand which outcrops in large cross-beds. The Coconino was first described in detail by the eminent Grand Canyon geologist Edwin McKee (1934) who later used it as a type example of an ancient eolian sandstone (McKee 1979). The bedding style of the Coconino is dominated by wedge-planar cross-beds; it has not been until recently, when Whitmore et al. (2011) reported scattered planar-beds throughout the formation, that any other bedding styles were widely reported in the Coconino. Whether the Coconino is observed at its southern margin near Pine (where it is about 300 m [984 ft] thick), or at the northern edge of the formation near the Arizona-Utah border (where it thins to only a fraction of a meter), the wedge-planar cross-bedding style is dominant and persistent. In central Arizona the cross-bedded portions of the Schnebly Hill Formation are transitional with the overlying Coconino. For the most part, the contact between the two formations is defined by color; changing from red in the Schnebly Hill to tan in the Coconino. The Toroweap Formation lies above the Coconino, but in places it intertongues laterally and vertically with the Coconino (Blakey and Knepp 1989). The Toroweap primarily consists of planar-bedded limestones, dolomite, sandstone, and gypsum which were deposited in a shallow ocean (Rawson and Turner-Peterson 1980). The Toroweap occasionally has a cross-bedded sandstone facies which is similar to that found in the Schnebly Hill and Coconino. In the Toroweap, the cross-beds are often interpreted as the product of coastal sand dunes (Rawson and Turner-Peterson 1980).
Here we report meters-thick, many tens of meters-long and extensive (>375 km2 [144.7 mi2]) occurrence of folds, many of which resemble parabolic recumbent folds (PRFs), in parts of the Schnebly Hill, Coconino Sandstone and Toroweap Formation cross-beds in the Sedona, Arizona area (Fig. 1). We will focus on deformation found in the Coconino Sandstone. Only two folded areas have previously been reported in these formations, both interpreted as eolian slumps: one from the Coconino in the Wupatki National Monument area (McKee 1979) and several from the Toroweap in the Oak Creek Canyon area (Rawson and Turner-Peterson 1980). The deformation we are describing in these formations is not vertically developed, but confined laterally to long individual cross-bed sets; it is quite different from the soft-sediment deformation features and convolute bedding commonly found in the Navajo Sandstone (Bryant and Miall 2010) and other types of deformation related to seismic processes (Alsop and Marco 2011; Owen, Moretti, and Alfaro 2011), which forms subsequent to deposition.
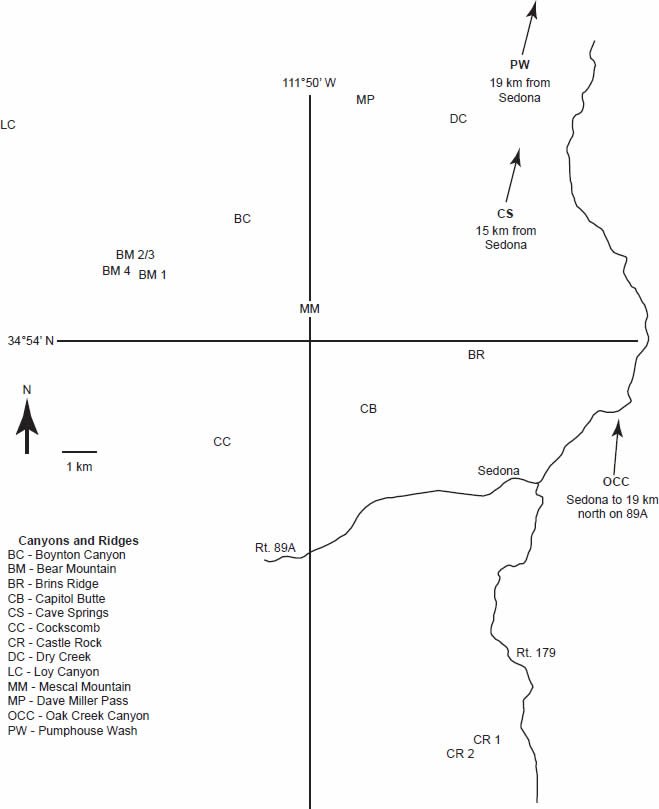
Fig. 1. Map of Sedona, Arizona area showing locations where we have found Type I and II deformation of cross-beds in the Coconino Sandstone, Schnebly Hill, and Toroweap Formations (deformation types illustrated in Fig. 2 and defined in the text).
Several authors have described various types of deformed cross-bedding in sandstones (Allen and Banks 1972; Doe and Dott 1980; Hendry and Stauffer 1975) and for the purposes of this report we define them as Types I, II, and III (Fig. 2).
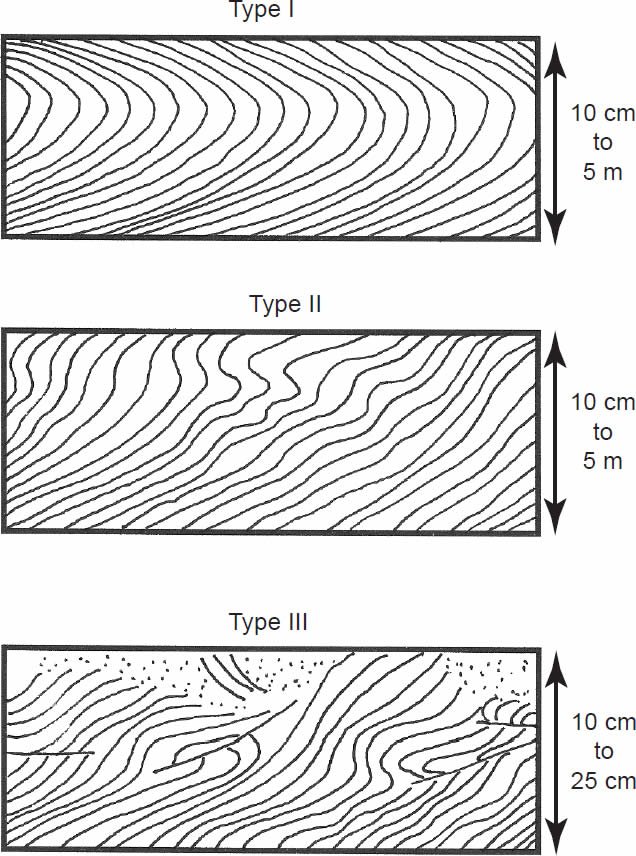
Fig. 2. The three primary types of deformation that occur in cross-bedded sandstone units (descriptions in text and drawings after several authors [Allen and Banks 1972; Doe and Dott 1980; Hendry and Stauffer 1975]). Note that the scale of eolian deformational features is rather small compared to those formed by subaqueous processes.
Type I (or parabolic recumbent) folds are single recumbent folds, typically each following a smooth parabolic curve, the axial plane of which is close to horizontal. The “mouth” of the fold opens down-current. The hinge may occur at any level within the deformed unit, but typically occurs near the middle or upper part of the bed. The stratification in the lower part of the bed is well defined, but stratification in the upper part of the bed becomes faint or blurred and sometimes impossible to detect. In less well developed examples, the upper strata are more steeply inclined but with no overturning. The top of the fold is truncated as in an angular unconformity or sometimes becomes doubly recumbent. Based on published laboratory experiments, literature review and field observations, these types of folds most commonly occur in beds from 0.1 m (0.32 ft) to about 2 m (6.56 ft) in thickness, with some even thicker. Small examples have been produced experimentally but only in subaqueous settings. All previously known field examples occur in subaqueous cross-bedded sandstones (see table in Wells et al. 1993). Sometimes sheath folds are associated with the recumbent folds (Foos 2003, Plate 3-B).
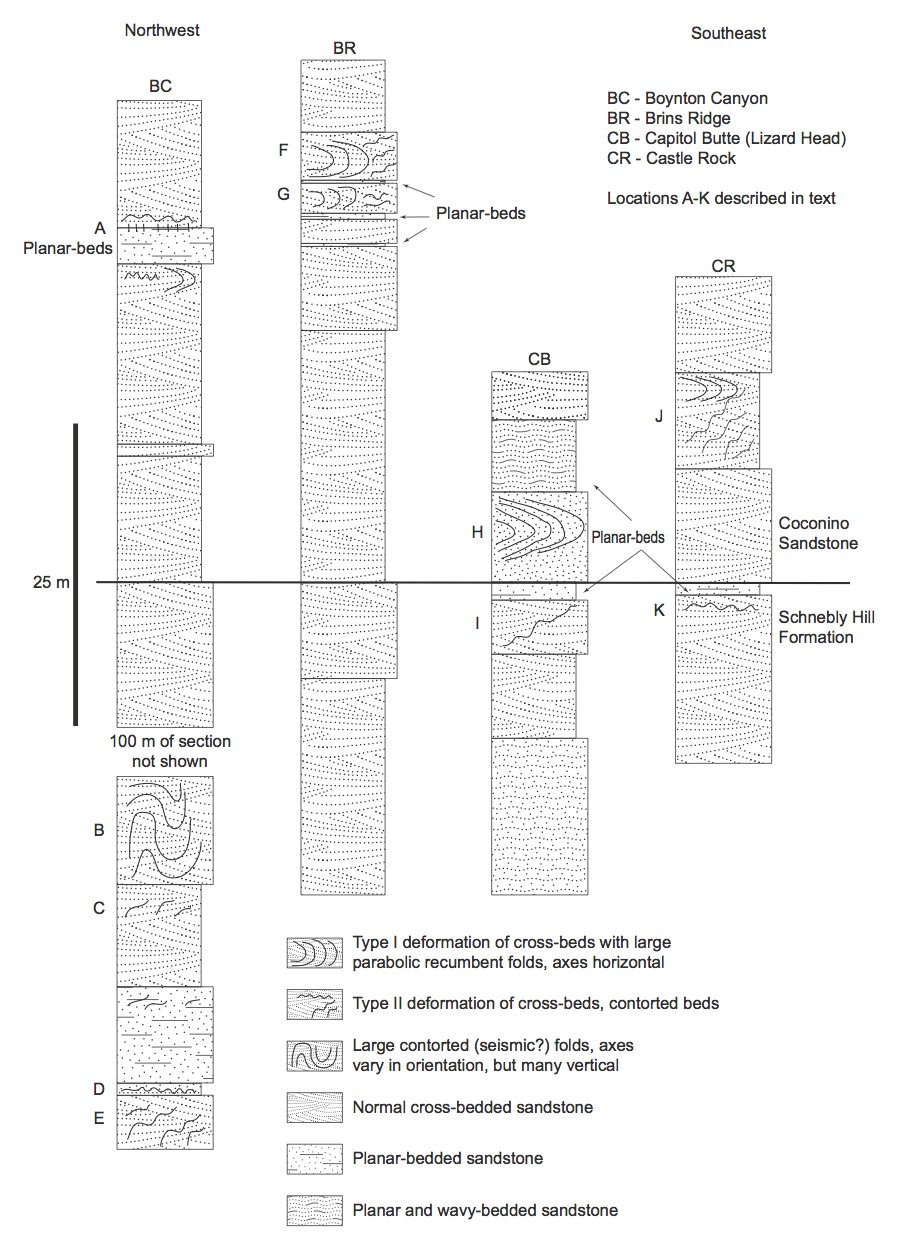
Fig. 3. Stratigraphic sections of four prominent folded areas in the Sedona, Arizona area. Deformation types described in text.
Type II ( or contorted) folds are numerous folds that differ in the size, shape, and attitudes of the axial planes. The largest and most complex folding (some of which may be disharmonic) occurs near the top of the cross-bed set. Near the bottom of the cross-bed set, less folding and deformation occurs. As in Type I folds, stratification in the upper part of the cross-bed set may be blurred or absent. Faulting is absent. Type I and Type II folds can occur together in the same cross-bed set showing a genetic relationship between the two types of folding. The deformation can be mild or rather complex. Beds occur in thicknesses from 0.1 to 5 m (0.32 to 16.4 ft).
Type III (or brecciated and faulted) folds include deformation structures that contain a mixture of overturned folds, thrust faults, “crinkly” bedding and structureless sand. This type of deformation is relatively small scale (0.1–0.25 m [0.32–0.82 ft]) compared to the other two types, and is only known to form in eolian settings from slumping of relatively cohesive (wet but not saturated) sand. McKee and Bigarella (1979) and McKee, Douglass, and Rittenhouse (1971) illustrate many types of these deformation structures based on experimental work and field studies of modern dunes.
Parabolic recumbent folds (PRFs) have been produced experimentally, but only in water-saturated sands (McKee, Reynolds, and Baker 1962a, 1962b) and have been observed in many modern and ancient subaqueous sandstones (Bhattacharya and Bandyopadhyay 1998; Coleman 1969; Cosentino 2007; Dott 1966; Hendry and Stauffer 1975; Jones 1962; Mazumder and Altermann 2007; McCormick and Picard 1969; McKee 1962; Reineck and Singh 1980; Robson 1956; Røe and Hermansen 2006; Rust 1968; Samaila et al. 2006; Stewart 1961; Wells et al. 1993). PRFs are thought to be impossible to form in sand that is not completely water-saturated; otherwise it is too cohesive to fold and faults are instead produced. Liquefaction of the sand is necessary in order to reduce cohesion and allow folding to occur (Allen and Banks 1972). In referring to these as “intraformational” PRFs we are borrowing terminology used by McKee, Reynolds, and Baker (1962a). Others have referred to them simply as “parabolic recumbent folds” (Doe and Dott 1980). Intraformational refers to deformation that occurs between undeformed beds.
It is not atypical for large cross-bedded sand units to display convolute bedding, soft-sediment deformation, liquefaction, and fluidization features. When deformation features occur in ancient eolian sandstones, they mostly have been attributed to groundwater saturation and subsequent fluidization and liquefaction well after deposition (Bryant and Miall 2010; Doe and Dott 1980; Horowitz 1982; Hurst and Glennie 2008). We have found some structureless bedding, pipes, and large deformed beds in parts of the Schnebly Hill Formation (below the Fort Apache Limestone in the Boynton Canyon section) and we recognize they are probably seismic liquefaction structures; but we are not focusing on those features here.
Field Observations and Methods
We have located multiple folded horizons and widespread occurrence of Types I and II deformation in the Schnebly Hill Formation and Coconino Sandstone in the Sedona area; one fold in the Coconino along Pine Creek Trail, near Pine, Arizona; and several folds in the Toroweap Formation in the National Forest north of Sedona (see Table 1). We also located and reexamined McKee’s (1979) Coconino fold in what is now Wupatki National Monument. We measured four sections in the Sedona area (Fig. 3) to see whether or not some of the features possibly correlated with each other. We have not found Type III deformation (characteristic of eolian deposits) in any of these formations, nor have any such features been reported of which we are aware. We have extensively examined the Coconino as part of a larger petrology project.
Table 1. Types and locations of some of the soft-sediment deformation features in the Sedona, Arizona area in the Schnebly Hill Formation (SH), the Coconino Sandstone (CS) and the Toroweap Formation (TF). See Fig. 1 for a map of fold locations. Fold types (Type I and II) are shown in Fig. 2 and described in the text. “WE” stands for possible water escape features including the “slits” we describe in the text. Fig. 3 shows the stratigraphy of the BC, BR, CB, and CR sections and the locations of cross-section labels A–J.
Fold Location | Coordinates | Formation | Fold Types | Notes |
---|---|---|---|---|
BC-1 Fig. 3 label A |
34.9307°N 111.8531°W |
CS | II | Photo in Fig. 4 (small-scale folding). |
BC-2 Fig. 3 label A |
34.9307°N 111.8531°W |
CS | WE? | Photo in Fig. 4 (“slits”). |
BC-3 | 34.9307°N 111.8531°W |
CS | II | Near Fig. 4 photo. About 1.5 m (4.9 ft) of deformation, below planar-beds. |
BC-4 Fig 3 label B |
34.9288°N 111.8535°W |
SH | Anticlines and synclines with vertical axes | Medium and large (up to 5 m [16.4 ft] tall) folds in at least one bed, 9 m (29.5 ft) total deformation, typical of seismically generated deformation, at least 0.5 km (0.31 mi) in length. Can be traced throughout Sedona area. |
BC-5 Fig. 3 label C |
34.9280°N 111.8528°W |
SH | II | Photo in Fig. 5. |
BC-6 Fig 3 label D |
34.9275°N 111.8530°W |
SH | homogenized | 1.0 m (3.2 ft)-thick zone with some pipes below, bedding in zone has been completely lost, probably seismic. |
BC-7 Fig. 3 label E |
34.5564°N 111.8537°W |
SH | II | Small- to medium-scale deformed cross-beds. |
BM-1 | 34.90172°N 111.8688°W |
CS | II | Series of medium folds in the Coconino. Runs along face for 60 m (196 ft). Sits directly above 0.5 m (1.6 ft) planar-bed. |
BM-2 | 34.9061°N 111.8785°W |
CS | II | Small-, medium- and large-scale folds along a cross-bedded face for >100 m (328 ft), sitting on a 2 m (6.5 ft)-thick planar-bed. |
BM-3 | 34.9052°N 111.8786°W |
CS SH |
II | One isolated large fold located 5 m (16.4 ft) below BM-3 and within cross-beds. |
BM-4 | 34.9032°N 111.8750°W |
CS/SH | II | One isolated fold located 360 m (1181 ft) SE of BM-3 at same altitude. |
BR-1 Fig. 3 labels F and G |
34.8953°N 111.7797°W |
CS | I II |
Described in text. Photos in Figs. 6-8, deformation zones at least 450 m (1476 ft) long. Longest continuous zone 170 m (557 ft) long. |
CB-1 Fig. 3 labels H and I |
34.8835°N 111.8161°W |
CS SH |
I, II II |
Photo in Fig. 10, 11 and 12. Type I and II in Coconino, Type II in Schnebly. Continuous folds in Coconino extend at least 50 m (164 ft). |
CC-1 | 34.8752°N 111.8583°W |
SH | WE | Water escape pipes, probably seismic deformation. |
CR-1 Fig. 3 labels J and K |
34.8024°N 111.7763°W |
CS SH |
II | Several medium- and large-scale folds at several locations in the Schnebly and the Coconino. Photo in Fig. 13. |
CR-1 Fig. 3 label J |
34.7932°N 111.7799°W |
CS | I | Several medium-scale folds widely scattered on ridge top about 500 m (1640 ft) west of CR-1. Photo in Fig. 14. |
CS-1 | 35.0052°N 111.7329°W |
TF | II | Two large folds originally described by Rawson and Turner-Peterson (1980). We are not certain of their location or that we found the folds originally described by them. |
DC-1 | 34.9572°N 111.7914°W |
SH | I | One isolated, large-scale recumbent fold beside one medium-scale fold. |
LY-1 | 34.9477°N 111.9302°W |
SH | II | Many scattered folds in different layers. |
MM-1 | 34.9077°N 111.8833°W |
SH | II | Medium-scale folding in one bed. |
MP-1 | 34.9609°N 111.8199°W |
CS | II | Medium-scale folding. |
PCT | 34.4414°N 111.4228°W |
CS | II | Small fold near bottom of Coconino along Pine Creek Trail, near Pine, Arizona. |
PW-1 | 35.0398°N 111.7202°W |
TF | I II |
Large-scale fold and medium-scale fold 10 m (32.8 ft) east of main fold. |
OCC | Oak Creek Canyon area | TF | I | Large recumbent fold described (but not pictured) by Rawson and Turner-Peterson (1980). |
WNM-1 | 3.2 km NE of Doney Crater | CS | I | Large-scale fold within Wupatki National Monument. Photo in Figure 15. Location not shown in Fig. 3. |
Well-developed PRFs were examined in the Sharon Conglomerate (sometimes referred to as the Sharon Formation) of northeast Ohio (see Wells et al. 1993) to gain some familiarity with how these types of folds occurred in other cross-bedded sandstones. Following is a description of some of the pertinent PRFs and other deformation features we have found in Arizona.
Boynton Canyon (Fig. 3, BC), Sedona, Arizona
We measured 207 m (679 ft) of section beginning in the Schnebly Hill Formation, about 170 m (557 ft) below the contact with the Coconino Sandstone. The location was on the northeast side of Boynton Canyon, approximately 11 km (6.8 mi) northwest of Sedona. All the cross-section labels refer to Fig. 3. Sections are described from top-down, since most of the features of interest occur in the Coconino.
Cross-section label A: A 3.0 m (9.8 ft)-thick, extensive planar-bedded sandstone is sandwiched between typical cross-bedded Coconino Sandstone. There is about 2.0 m (6.5 ft) of Type II deformation just below the planar-beds. The upper contact with the planar-beds has regularly spaced (0.5–1.0 m) (1.6–3.2 ft) vertical “slits” 0.2 to 0.5 m (0.6 to 1.6 ft) long (Fig. 4). The slits begin in the planar-bedded sandstone and end in the cross-bedded sandstone. The upper ends of the slits are associated with Type II deformation in the cross-bedded sandstone above. The slits are oriented perpendicular to cross-bed strike (N70°W) at approximately N160°W. Cross-bed dips range from 19 to 25° to the southeast. The planar-bed and the slits can be traced for at least 300 m (984 ft) along the ridge. The slits can be observed on both sides of the ridge. The soft-sediment deformation does not occur everywhere, but where it does occur, it is directly above the planar-bedded horizon with 0.1 to 1.5 m (0.3 to 4.9 ft) of the cross-beds being deformed.
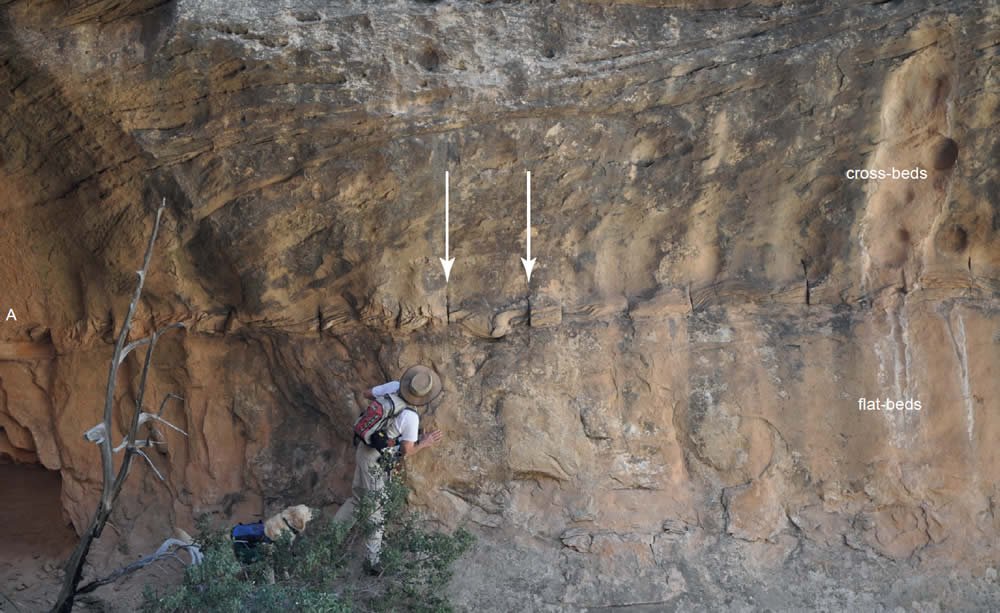
Fig. 4. Type II folding and “slits” above a 3 m (9.8 ft)-thick planar-bedded zone in the Coconino Sandstone, Boynton Canyon, cross-section label A and BC-1, 2, 3 (in Table 1). The “slits” begin in the planar-bedded zone and end in the cross-beds above. The slits are perpendicular to the strike of the cross-beds. A large Type II (I?) fold occurs out of view to the right of this picture, on a cliff face that is difficult to observe, deforming about 1.5 m (4.9 ft) of section, just below the planar-bed.
Cross-section label B: Thick folded (convolute) zone in Schnebly Hill Formation, about 9 m (29.5 ft) thick. It is difficult to tell whether the unit began as cross-bedded or planar-bedded since it is so deformed everywhere we have access to it. Many of the folds have vertical axes, and others are overturned (but not like Type I deformation). The deformation can be traced for 0.5 km (0.3 mi) along the face of the outcrop and across the valley. Deformation is primarily in the 9 m (29.5 ft) bed, though small areas of deformation are found at four different altitudes from this area to the top of the Schnebly Hill Formation. This unit appears to be typical of seismic deformation of water-saturated sand and is different from the PRFs that we are primarily concerned with in this paper.
Cross-section label C: An 8.5 m (27.8 ft) section of Schnebly Hill containing medium- to large-scale cross-beds with some planar-beds near the base and middle of the section. Some of the cross-beds in the top half of the section exhibit Type II deformation (Fig. 5). Laterally, the cross-beds grade from normal cross-beds into deformed cross-beds and then back into normal cross-beds. Deformation occurs down cross-bed dip. The undeformed cross-beds had higher dips than average for the Schnebly Hill and Coconino. The cross-bed dips were at 27°, where normally the Coconino cross-beds average about 20° (Emery, Maithel, and Whitmore 2011). The tops of several pipes, up to 0.3 m (0.98 ft) in diameter could be observed at two different altitudes in this section. They do not appear to be physically associated with the Type II deformed cross-bedding we are describing here.
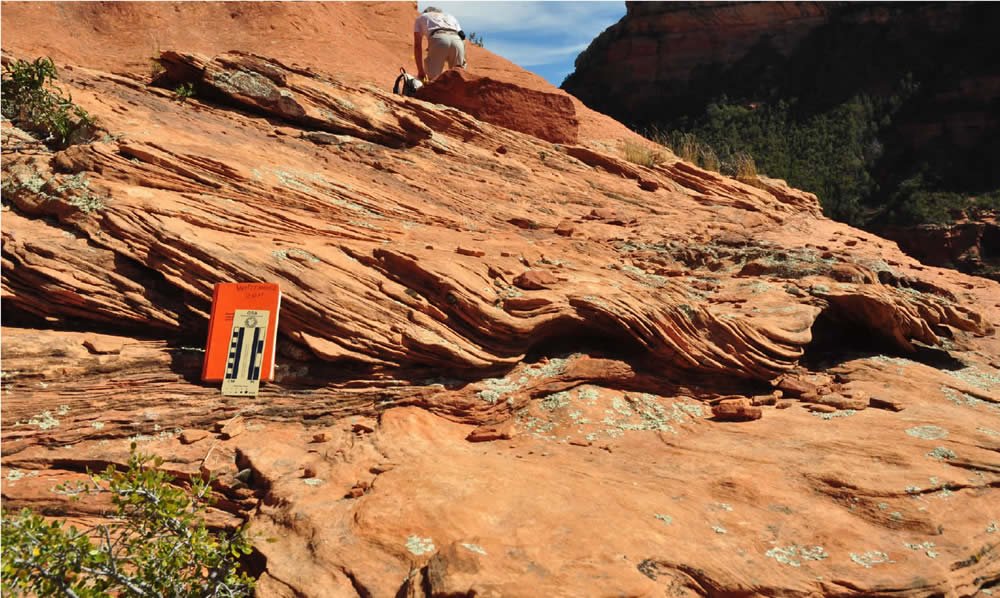
Fig. 5. Folding in the Schnebly Hill Formation, Boynton Canyon, cross-section label C in Fig. 3, BC-5 (in Table 1). Notice the normal cross-bedding (dipping 27°) in the left of the photo behind the field book and how it transitions to deformed bedding towards the right of the photo. Deformation occurs down-dip. Current was flowing left to right. Photo scale in picture is 0.1 m (0.3 ft).
Cross-section labels D and E: Cross-section label E is a 4.5 m (14.7 ft)-thick unit with small- to medium-scale cross-beds with some small Type II deformation features about 2 m (6.5 ft) from the base. The unit is capped by a 1.0 m (3.2 ft)-thick structureless sandstone (cross-section label D) that has several pipes at its base (up to 0.2 m [0.65 ft] in diameter, at least several meters long) which probably represent a liquefied source for the structureless sand in D. There is some minor Type II deformation between labels D and C.
Brins Ridge (Fig. 3, BR), Sedona, Arizona
Cross-section labels F and G: We measured 130 m (426 ft) of section starting about 85 m (278 ft) below the contact with the Coconino, which is transitional in this area. Just below the top of Brins Ridge (about 3 km [1.8 mi] north of Sedona) are two deformed zones (Type I and II), each having thin planar-bedded sandstone horizons below them. The deformed layers can be traced (discontinuously) for about 450 m (1476 ft) along the ridge top. Bed G is about 2.5 m (8.2 ft) thick and rests on a 0.50 m (1.6 ft)- thick planar-bedded sandstone. Bed F is about 4 m (13 ft) thick and rests on a 0.25 m (0.8 ft)-thick planar-bedded sandstone. Sometimes the planar-bedded sandstones pinch in and out along the length of the outcrop (In vertical exposures sometimes up to four thin planar-bedded horizons can be seen). Both units (F and G) contain Types I and II deformation (Figs. 6, 7, and 8). There are two sets of PRFs in Fig. 6. In the lower folded bed of Fig. 6 (the cliff face is almost parallel with dip) the transition can be seen from normal cross-bedding (on the right) to PRFs (on the left). To the far left, the fold begins to become doubly recumbent. It is very similar to a PRF we found in northeast Ohio (Fig. 9; Wells et al. 1993). The strike of the cross-beds is N130°W and the dip is 22° southeast. As in the other sites, cross-bed recumbent folding and deformation occurs down-dip. In the upper deformed cross-bed unit, the dip is slightly different and the beds are a bit more contorted; but deformation still occurs down-dip. Throughout the length of the ridge, both types of deformation fade in and out with normal cross-beds also in both layers. The longest set of recumbent folds was traceable for about 170 m (557.7 ft) along the ridge top (unit F). Fig. 7 shows Type I deformation in both of the deformed beds, and Fig. 8 shows Type II deformation in each of the deformed beds. Single recumbent folds occur in about 1.0 m (3.2 ft)-thick beds (as in Fig. 7). In thicker deformed beds the deformation can be more complex; with contortions and loss of laminae increasing towards the tops of the beds, as is typical in other described PRFs (Allen and Banks 1972). In areas along the southeast end of the ridge, the upper deformed zone (F) can be walked upon.
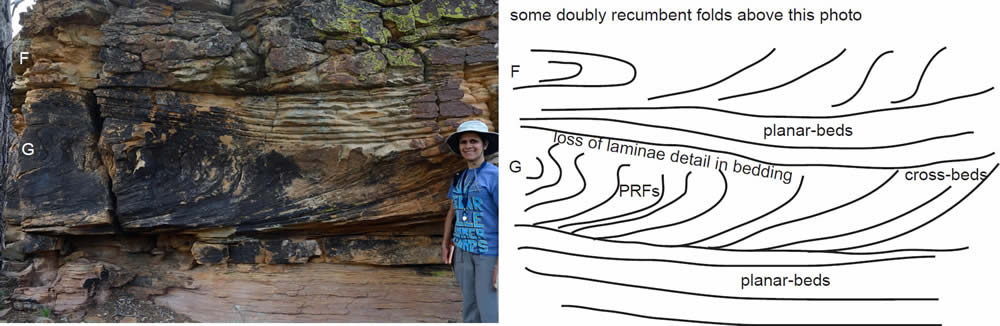
Fig. 6Two parabolic recumbent folds in the Coconino Sandstone along Brins Ridge, locations F and G, BR-1 (in Table 1). The lower fold is more easily seen in the photo than the upper one (from this angle). The photo was taken at a slightly oblique angle because of vegetation at the photographer’s back. Planar-beds separate the two deformed horizons. Current flowed from right to left. In the lower set of folds (cross-section label G in Fig. 3) note how the bedding changes from right to left. At the right they are steeply dipping cross-beds which change into parabolic recumbent folds towards the left. Deformation is more severe to the far left where the fold becomes doubly recumbent. Laminations are lost near the tops of the folds where they are also erosionally truncated by planarbeds. Examples similar to this (cross-beds transitioning into parabolic recumbent folds) were found in the Sharon Conglomerate of northeastern Ohio (see Wells et al. 1993 and Fig. 9).
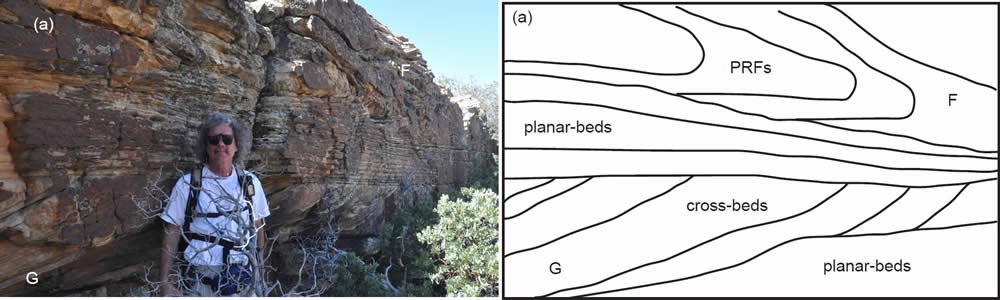
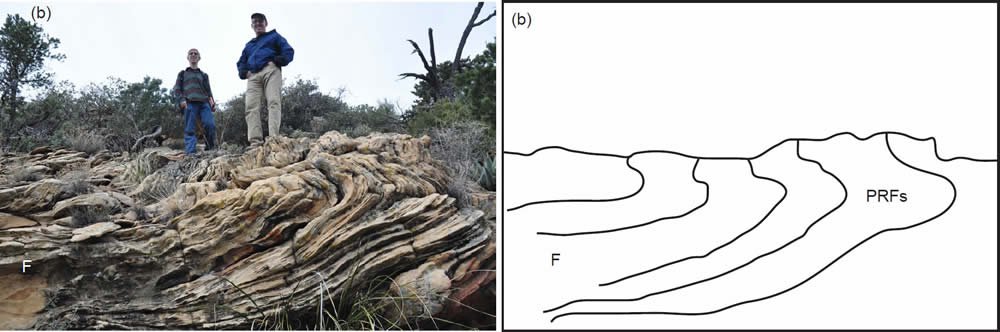
Fig. 7. (a) Planar-beds and a parabolic recumbent fold, cross-section label F of Fig. 3, BR-1 (in Table 1). The parabolic recumbent fold is above the planar-beds and is several meters to the right of the previous figure (Fig. 6). Current direction was from right to left. (b) The continuation of folded zone F, about 50 m (164 ft) to the northwest of the fold in Fig. 7(a). Again, flow is from right to left. This folded zone (F) can be traced for about 170 m (557 ft).
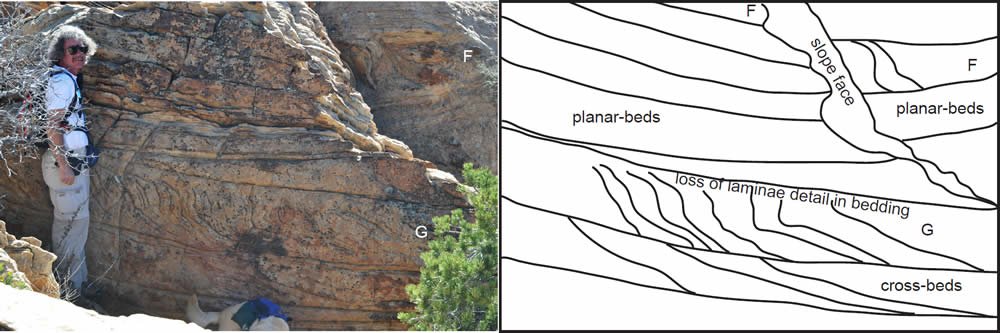
Fig. 8. Cross section labels F and G of Fig. 3, BR-1 (in Table 1). Cross-section labels F and G often change from cross-beds to Type I folds to Type II folds. In this photo, both beds are exhibiting Type II folds.
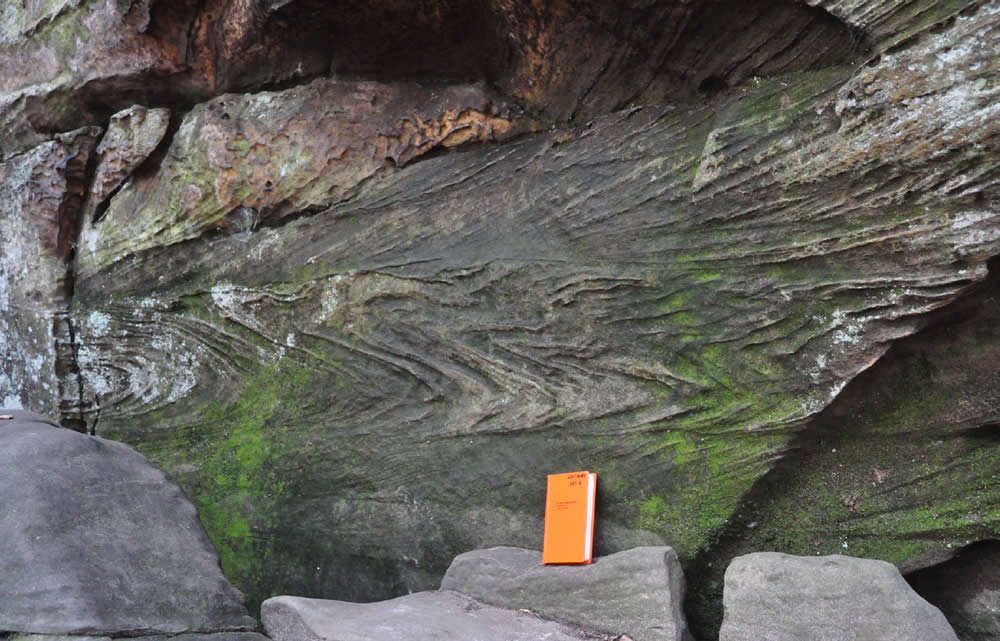
Fig. 9. A doubly recumbent PRF from the Sharon Conglomerate, Cuyahoga Valley National Park, near Peninsula, Ohio. The field book is about 0.2 m (0.65 ft) tall. These folds were described by Wells et al. (1993). We found a similar fold in the Coconino Sandstone, illustrated in Fig. 6.
Capitol Butte (Fig. 3, CB), Sedona, Arizona
Capitol Butte (with a rock ridge locally known as “Lizard Head” at its west end) is a prominent landmark about 3 km (1.8 mi) northwest of Sedona. Near the base of the Coconino Sandstone (cross-section label H) is a large PRF (Figs. 10, 11, and 12) about 5.0 m (16.4 ft)-thick, part of which is contained within the “Lizard Head.” The fold extends continuously east for at least 50 m (164 ft) along the outcrop to the east, maintaining its parabolic shape. The parabolic folds along the top of the ridge can be seen from a distance on both the north and south sides of the ridge. Further to the east, normal cross-bedding returns with Type II deformation within the cross-beds. Near the top of the fold, the laminations in the sandstone are more poorly defined. Below the folded zone there is a 3.0 m (9.8 ft)-thick cross-bedded unit which lies on top of a 1.5 m (4.9 ft)-thick planar-bedded zone. In this area, the Schnebly Hill/Coconino contact is transitional; we put the contact at the top of the planar-bedded zone. A 1.5 m (4.9 ft)-thick cross-bedded unit lies unconformably on top of the fold, and that is followed by a 6.0 m (19.6 ft)-thick planar-bedded zone. We made strike measurements in two places along the outcrop of the large fold at the west end of the ridge (N33°E and N60°E). Strike was difficult to measure in the fold because of the sheer nature of the outcrop and possibly curvature of the fold hinge; we think the N60°E measurement is probably closer to reality for most of the fold. Cross-bed strike below the fold was N70°E with a dip of 19° to the south. The overall geometry of the fold between Fig. 10 and Fig. 11 is like a sheath fold (which is probably why our strikes are variable); similar folds have been described associated with PRFs in the Sharon Conglomerate (see Plate 3-B in Foos 2003).
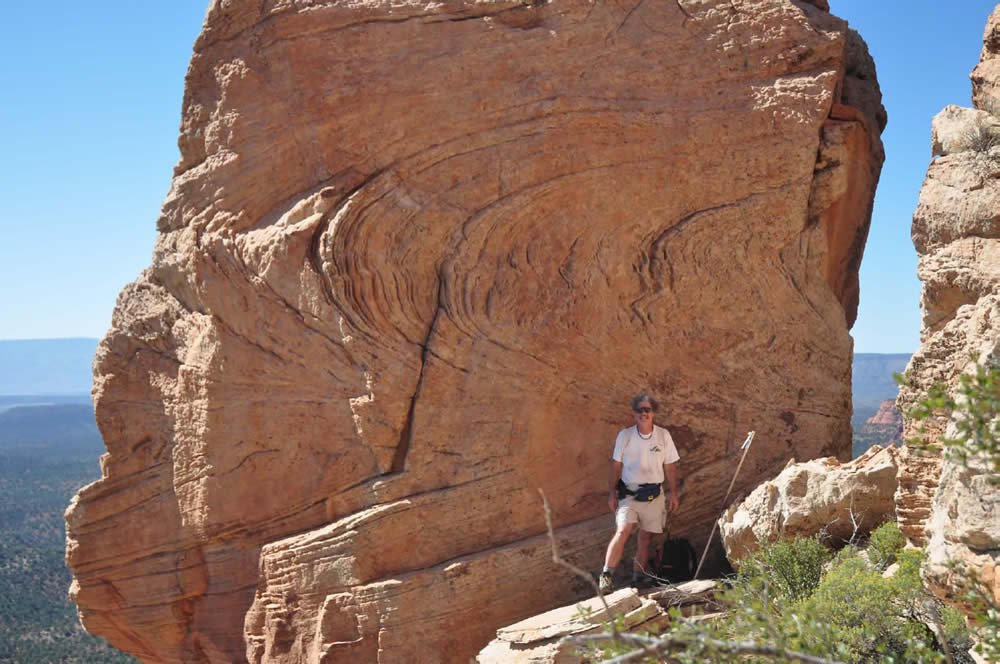
Fig. 10. A large parabolic recumbent fold (Type I deformation) occurring in the “Lizard Head” in the Coconino Sandstone of Capitol Butte, cross-section label H, CB-1 (in Table 1). The deformed zone is about 5.0 m (16.4 ft) thick. The laminations of the sandstone become less distinct near the top of the deformation. The fold is erosionally truncated by a 1.5 m (4.9 ft) cross-bedded zone at its top (which is difficult to see in Figs. 11 and 12). The fold extends about 50 m (164 ft) to the right (see Fig. 11 and 12). The Jacob’s staff is 1.5 m (4.9 ft) in length. Fold characteristics to the right of this photo suggest that it is part of a larger “sheath” fold (see Plate 3-B of Foos 2003). Wells et al. (1993, p. 73) state that many PRFs take the form of a concentric oval when viewed end-on.
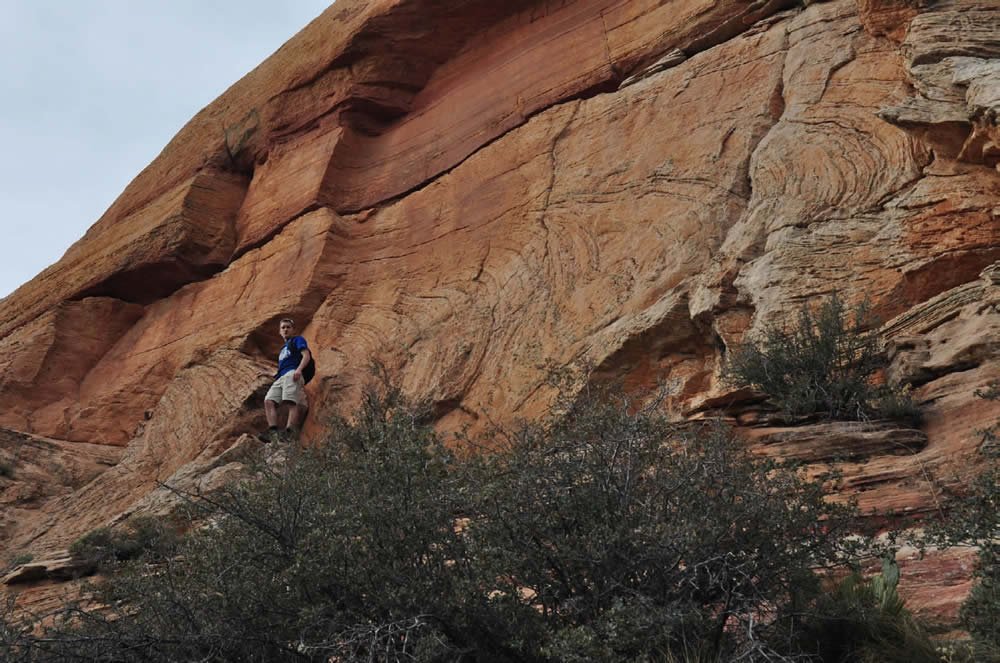
Fig. 11. Part of the large parabolic recumbent fold shown in Fig. 10, cross-section label H, CB-1. The zone of deformation is about 5.0 m (16.4 ft) thick and about 50 m (164 ft) long. Current flowed approximately right to left (cliff is not parallel to dip). Note how the laminations become less distinct near the top of the fold. Below the fold there is a 3.0 m (9.8 ft)-thick cross-bedded unit (hidden in this upslope view) which lies on top of a 1.5 m (4.9 ft)-thick planar-bedded zone (behind the vegetation in this photo). A 1.5 m (4.9 ft)-thick cross-bedded unit lies unconformably on top of the fold (also difficult to see in this view), and that is followed by a 6 m (19.6 ft)-thick wavy- and planar-bedded zone (seen in the upper part of the rock section).
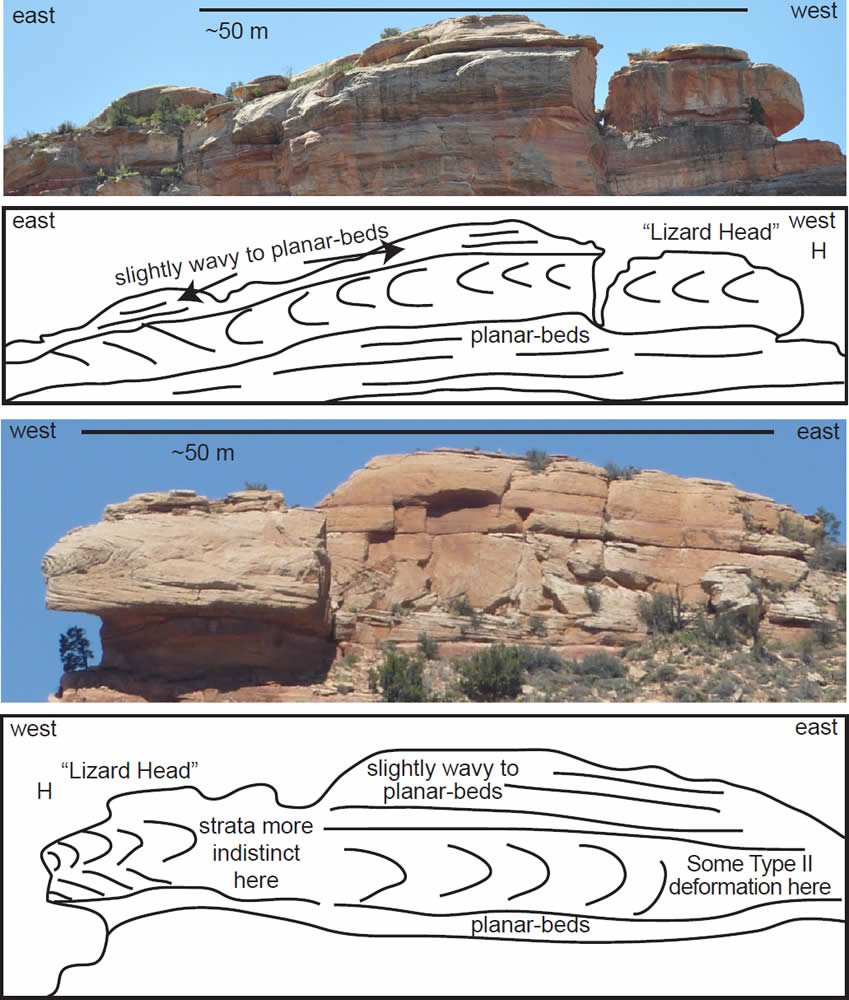
Fig. 12. The opposite sides of “Lizard Head” ridge showing that the folds extend through the ridge (CB-1). The folds extend for about 50 m (164 ft) along the ridge.
There is also some Type II deformation within the Schnebly Hill Formation at this location (Fig. 3, cross-section label I). Those folds occur through a 4.5 m (14.7 ft)-thick zone of large-scale cross-beds.
Castle Rock (Fig. 3, CR), Sedona, Arizona
We measured about 142 m (465.8 ft) of section at Castle Rock, beginning about 115 m (377 ft) below the base of the Coconino. Castle Rock is about 8 km (4.9 mi) south of Sedona. There is a 1.0 m (3.2 ft)- thick planar-bedded sandstone that marks the top of the Schnebly Hill Formation. Type II deformation begins in the Coconino cross-beds about 8.0 m (26.2 ft) above the base and continues for about 8.0 m (26.2 ft) through the section. Type II deformation is the most common here (CR-1, Fig. 13), but there are some well-developed examples of medium-scale Type I deformation about 500 m (1640 ft) to the west (CR-2, Fig. 14). In the CR-1 area, there are a few scattered Type II folds in the Schnebly Hill (Fig. 3, label K).
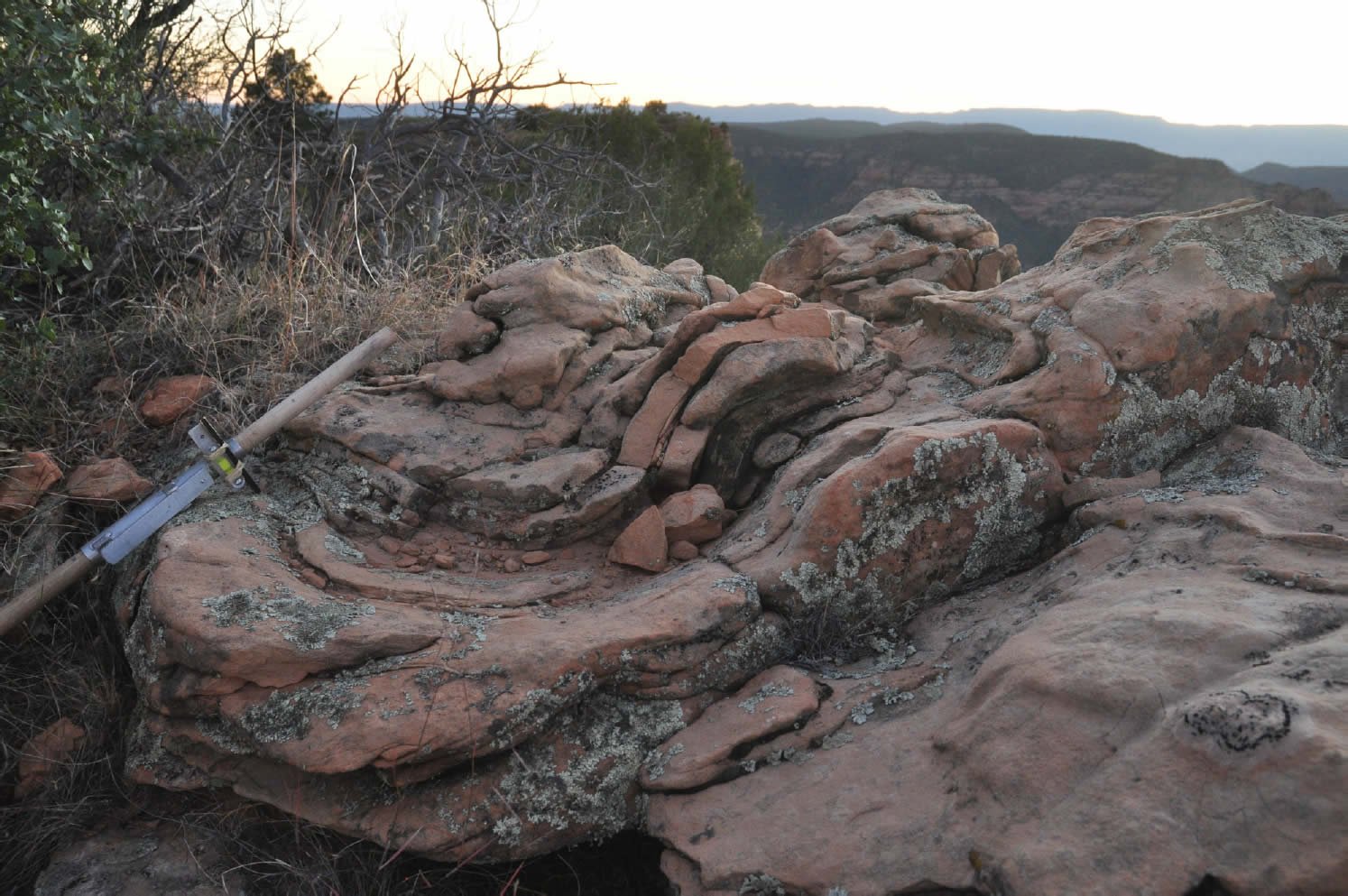
Fig. 13. Type II deformation at Castle Rock (CR-1), cross-section label J in Fig. 3. About one-half of the 1.5 m (4.9 ft) Jacob’s staff is showing in the left side of the photo.
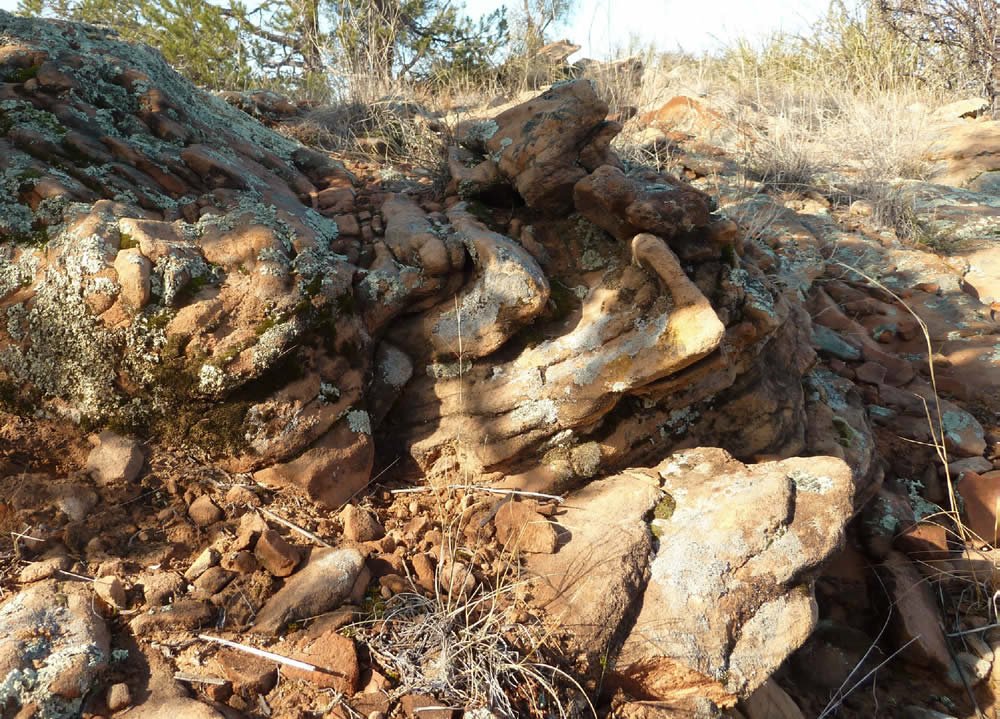
Fig. 14. A parabolic recumbent fold (Type I) at Castle Rock (CR-2), cross-section label J. The zone of deformation is about 1.0 m (3.2 ft) thick. Current flow was from right to left. About 0.5 m (1.6 ft) thickness of rock is deformed in this photo.
Pine Creek Trail (PCT), Pine, Arizona
A small exposure of Type II deformation occurs near the bottom of the Coconino section along the Pine Creek Trail, near Pine, Arizona. Only about 0.5 m (1.6 ft) of cross-bedding was deformed. The deformation was only noted along the trail; it was not searched for off-trail.
Toroweap folds
Folding in the Toroweap Formation north of Sedona was mentioned by Rawson and Turner-Peterson (1980, p. 349) but the locations specified in the paper were only general in nature. We have found a folded area near Cave Springs in Oak Creek Canyon that may be one of their outcrops (OOC-1). A more significant fold was discovered in the area of Pumphouse Wash (PW-1), about 18 km (11.18 mi) NNE of Sedona. The fold deforms about 3 vertical meters (9.8 ft) of strata. It continues along the rock face for about 10 m (32.8 ft). Another small fold occurs about 4 m (13.1 ft) to the east of the main folded area. The fold is within cross-bedded strata with a strike of S8°W and a dip of 27°S. The hinge of the fold could not be directly measured, but appears to be perpendicular to dip.
Wupatki National Monument, Fig. 15
McKee (1979) included a photograph of a fold in the Coconino describing it as 3.2 km (1.9 mi) northeast of Doney Crater, north of Flagstaff, Arizona. Billingsley, Priest, and Felger (2007) and personal communication with Billingsley (2011) suggests the mapped area where the fold is located is Toroweap Formation. However our field check indicated the fold is in the upper 10 m (32.8 ft) of the Coconino, exposed by a small fault in the area. The fold deforms about 3 m (9.8 ft) of southerly dipping cross-strata and is clearly recumbent in nature. There were no faults or other typical eolian deformation (Type III) features associated with the fold. The strike of the hinge of the fold was N9°W.
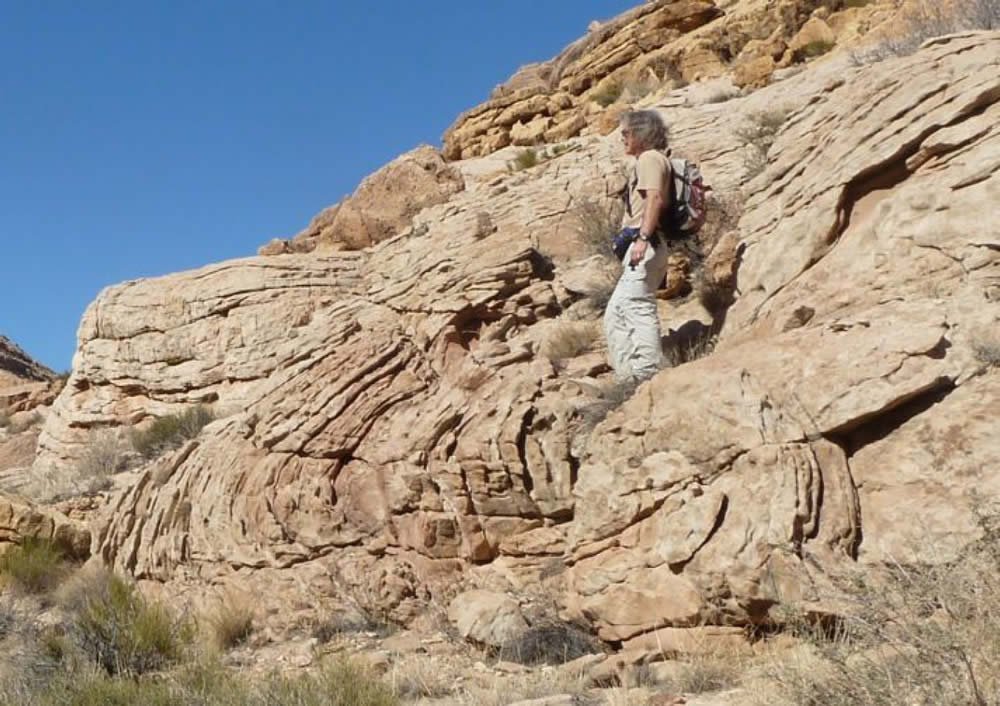
Fig. 15. We located the fold McKee (1979) interpreted as an eolian slump in the Coconino Sandstone, Wupatki National Monument, Arizona (WNM-1). Field evidence suggests it is a large parabolic recumbent fold. The fold occurs near the top of the Coconino (Toroweap is in the top of the photo).
Other observations
The purpose of this paper is primarily to describe the folds that we have found in the Permian sandstones near Sedona. However, we briefly report here some other data that we have collected, particularly in the Coconino. Since McKee (1934), little else other than stratigraphic work has been done on the Coconino. In our studies, we have extensively sampled outcrops of the Coconino throughout Arizona, primarily for petrographic analysis. In our studies we have noticed the prevalence of primary current lineation on cross-bed foresets in nearly every outcrop; the presence of trace amounts of muscovite in nearly every thin section examined (>250); the presence of dolomite ooids within the cross-beds at several locations in the northern part of the outcrop area; the presence of dolomite beds, cements, and clasts at many locations; the sub-rounded to sub-angular nature of the quartz sand grains; the moderately to poorly sorted nature of the sand grains; K-feldspar grains that are more angular than the surrounding quartz grains; cross-bed dips much less than the angle of repose of dry sand (on average, 20°); the absence of tongue-shaped avalanche scars (as seen on the foresets of modern dunes); and the lack of small-scale deformation features as commonly seen in modern dunes (McKee, Douglass, and Rittenhouse 1971). Our petrographic data is documented in Whitmore et al. (2014).
Discussion
The mechanism of parabolic recumbent fold formation
Allen and Banks (1972) reviewed various hypotheses for the formation of PRFs under both subaerial and subaqueous conditions. These included downslope sliding of sediment in subaerial conditions. They found this type of movement inadequate to explain the first two types of folding (Types I and II, Fig. 2), especially when it occurred at large scales, but quite sufficient to explain the third type (Type III, Fig. 2), which produces smaller scale structures (small, laminae-scale faults and folds). At least nine types of these small-scale structures (sketched in 15 × 10 cm [5.9 × 3.9 in] boxes) have been documented from modern eolian settings (McKee and Bigarella 1979; McKee, Douglass, and Rittenhouse 1971). Several hypotheses have been put forward to explain the origin of PRFs under subaqueous conditions. Allen and Banks (1972) and Wells et al. (1993) have developed the most comprehensive models.
As summarized by Allen and Banks, a number of authors have favored recumbent folding due to the frictional drag of a sediment-laden current. But a water current alone may not be enough to cause folding; momentary liquefaction of the sediment probably needs to occur as well. Allen and Banks favored this idea and developed a theoretical model for the formation of PRFs. According to them, the shear force necessary to deform the strata could occur with a strong current flowing over a bed that was liquefied by seismic activity. As the sand was liquefied, the current contorted the bed in the down-dip direction. Experiments by Owen (1996, p. 290) “conclusively demonstrated that simple recumbent-folded cross-bedding is generated by tangential shear acting on a liquefied bed, and that sufficient shear can be provided by an aqueous current.” Owen generated liquefaction by performing his experiments on a shaker table.
Seismic activity may not always be responsible for liquefaction; other triggers have also been implicated. Some PRFs have formed in areas that did not experience seismic activity. Hendry and Stauffer (1975), who studied folds from Saskatchewan, Canada, argued that PRFs can be made by strong sediment-laden currents, without seismic activity. This also appears to be the case with modern folds formed in the Brahmaputra River sands (Coleman 1969). PRFs are so abundant in some cross-bedded sands that it is unlikely that strong syndepositional earth tremors caused all the folding, although earthquake activity could still be invoked for some folds. Thus, the formation of some folds by strong sediment-laden currents alone seems to be a reasonable explanation (Wells et al. 1993). McKee, Reynolds, and Baker (1962a) produced recumbent folds in the laboratory with strong sediment-laden currents. Formation of recumbent folds by shearing of a tangential current is supported by thin-section study as well (Yagishita and Morris 1979), but perhaps other mechanisms that include liquefaction of the basal sediment layer are possible.
Røe and Hermansen (2006) have suggested that recumbent folding can take place during changes in flow regime. They argued that cross-strata formed in the dune/plane-bed transition may be deformed as the flow regime momentarily changes to plane-bed stage, causing liquefaction at the dune front. The current then becomes sediment-laden (due to the liquefied sand), causing shear forces to deform the beds below.
Another possible mechanism suggested for liquefaction of bottom sediments is cyclic loading by sudden changes in the depth of the water column by waves (Molina et al. 1998; Owen and Moretti 2011) and even tides (Greb and Archer 2007). These mechanisms could potentially cause liquefaction and parabolic recumbent folding during deposition of cross-bedding in a subaqueous setting. Wells et al. (1993) suggested that one mechanism for deformed beds in the fluvial Sharon Conglomerate might be a sudden increase in water depth during a flash flood. It seems mechanisms like this might also be considered for deformation in the cross-beds of other rivers and deltas including the Brahmaputra River (Coleman 1969), the Mississippi River delta (Coleman and Gagliano 1965), Coos Bay Delta (Dott 1966), and the Colorado River (McKee 1938). PRFs have been observed in shallow marine sandstones of India (Mazumder and Altermann 2007), showing that these features can occur in settings other than fluvial ones.
The formation of parabolic recumbent folds in the Coconino
Considering Fig. 3, it does not appear that the folds (Types I and II) or the planar-beds correlate from section to section, even in the case of the two sections that are relatively close to each other (BR and CB). The folds appear to occur at different horizons within each of the sections. The deformed zones appear and disappear within individual cross-bed sets along the same horizon. The features do not show any vertical development, which is often the case when seismic activity causes liquefaction or fluidization; fluid escape often causes vertical zones of deformation in the strata above the main zone of deformation. These observations can probably rule out seismic origin for most of the features. The only features that we think might be seismic are those described in the Schnebly Hill Formation (BC, cross-section labels B and D). We have been able to trace the deformed strata layer B throughout the Sedona area indicating a widespread event that affected the entire area. On the other hand, PRFs tend to be more localized phenomena that fade in and out along the outcrop.
Several lines of evidence suggest the folding took place while the sand was water-saturated (similar arguments were set out by Hendry and Stauffer [1975]). Firstly, Rettger (1935) found that significant folding does not occur in dry sand, but only water-saturated sand. McKee, Reynolds, and Baker (1962a, 1962b) found similar results in their experiments. Secondly, in their experiments and observations of deformation in modern eolian sands McKee, Douglass, and Rittenhouse (1971) found that nine types of deformation structures typically occur in dry sand (rotated plates and blocks, stair-step folds and normal faults, stretched laminae, warps, (gentle folds), drag folds and flames, high-angle asymmetrical folds, overturned folds and overthrusts, break-apart laminae and breccias, fade-out laminae). All of these structures are small-scale, <0.25 m (0.82 ft), often less than 0.1 m (0.32 ft) in size. Faulting and suturing of laminae is common in dry sand, but does not typically occur in water-saturated sands. In Fig. 2, this is Type III deformation. These small-scale features do not occur in the folded horizons that we are describing, nor do we know of any reports of them in the Coconino literature. Even in modern wet or damp eolian sands, recumbent folds of the type we are describing are absent. McKee and Bigarella (1979) illustrate some recumbent folds and thrust features from modern dunes; but these features are mostly laminae-scale deformations riddled with small faults and are not comparable in shape or scale to those we are describing from the Sedona area (their sketches of these features are in 15 × 10 cm (5.9 × 3.9 in boxes). Thirdly, these types of folds (PRFs) are well-known from modern and ancient subaqueous settings (more than a dozen examples were cited in the introduction) and completely unknown in modern eolian settings. In fact, Doe and Dott (1980, p. 808) argued that if PRFs were present in the Navajo Sandstone it would support subaqueous deposition for that formation, a topic that was being hotly debated in the literature at that time. Fourthly, these types of folds have been produced in the laboratory, but only in water-saturated conditions; dry sand and wet sand gave completely different results (McKee, Reynolds, and Baker 1962a). Fifthly, the folds in the Sedona area remain consistent in shape and size over great lengths of the outcrop within single beds with unconformably truncated tops. One set of intraformational folds was traced down-dip on Brins Ridge for 170 m (557 ft) and another set on Capitol Butte was traced for 50 m (164 ft). We think this is impossible to explain from the local slumping of dry or even damp sand dunes. Sixthly, transitions from cross-beds to folded beds can be found and are similar to those recognized in subaqueous deposits like the Sharon Conglomerate of northeastern Ohio (Wells et al. 1993).
We think there is good evidence that the folds are penecontemporaneous with the deposition of the individual cross-bed sets. Several observations support this conclusion. First, the deformation is intraformational. In other words, the deformed cross-beds are single beds which are truncated by overlying planar-beds or cross-beds in every case. The deformation does not grade into the overlying or underlying units as it might if groundwater movement or seismic activity had caused the deformation. Units above and below the deformed zones are typically unaffected by deformation, even when several units are successively folded (as in Fig. 6). Second, the planar-lying axial planes of the recumbent folds indicate that a force was necessary to rotate and horizontally translate the strata. This force had to be applied before successive layers were deposited on top of the deformed bed. Third, the folds are oriented in the same directions as the cross-bed dips, indicating that deforming forces in the same direction as cross-bed migration must have caused the folding. Fourth, examples can be found where cross-beds transition into beds that have been folded (Fig. 6). Single deformed beds, which transition into cross-beds have been traced for 170 m (557 .7 ft) on Brins Ridge and for 50 m (164 ft) on Capitol Butte (Figs. 6–8, 10–12). The folds are too long to be explained by a slumping dune.
There are four theories of PRF formation: 1) Strong sediment laden currents deform cross-beds (Coleman 1969; Hendry and Stauffer 1975; McKee, Reynolds, and Baker 1962a; Wells et al. 1993). 2) Strong currents deform cross-beds coincident with seismic activity (Allen and Banks 1972). 3) Strong currents deform cross-beds during changes in flow regime (Røe and Hermansen 2006). 4) Strong currents deform cross-beds coincident with sudden changes in water depth (Molina et al. 1998; Owen and Moretti 2011; Wells et al. 1993). Based on theoretical work by Allen and Banks (1972), an episode of brief liquefaction seems to be necessary to form at least the larger sets of PRFs. The first idea, unlike the last three, does not require liquefaction in order for PRFs to form.
We like the suggestion by Røe and Hermansen (2006) that recumbent folding can take place during changes in flow regime. They argued that cross-strata formed in the dune/plane-bed transition could be deformed as the flow regime momentarily changed to plane-bed stage, causing liquefaction at the dune front. The current then becomes sediment-laden (due to the liquefied sand), causing shear forces to deform the beds below. We do not know if this is the precise mechanism for the deformation of the cross-beds in the Sedona area, but we think it must be seriously considered. Planar-beds are extremely rare in the Coconino, with more occurring in the Sedona area than anywhere else (Whitmore et al. 2011). The close association of the Coconino planar-beds with the deformation structures we have found may indicate that currents are fluctuating back and forth between flow regimes. Everywhere we have found planar-beds in the Sedona area, deformed Coconino can be found in the vicinity.
Of course, the suggestion that the Coconino was deposited subaqueously will be an “outrage” (Davis 1926) to some. However, several previous workers have suggested subaqueous deposition for at least parts of the Coconino Sandstone and Schnebly Hill Formation. When McKee wrote his monograph in 1934, he suggested that part of the Coconino was subaqueous (see pp. 79 and 110), referring to planar Coconino beds that can be found at the transitional contact between the Hermit and Coconino along Tanner Trail in the Grand Canyon. Fisher (1961, p. 81) thought the Coconino was marine in the area of the Shivwits Plateau because of the transitional nature with marine Toroweap. Brand (1979) and Brand and Tang (1991) suggested that the cross-bedded portion of the Coconino was subaqueously deposited because of the unusual characteristics of vertebrate tracks on foresets that were difficult to explain in dry or damp subaerial sand (but see also the discussion of Lockley [1992] and Brand [1992]). Lundy (1973) came to the conclusion that the Coconino was deposited by subaqueous sand waves based upon his study of the petrology. Visher (1990, pp. 210–211) supported this view as well. Peirce, Jones, and Rogers (1977) thought that the Coconino in east central Arizona was marine because of the planar-bedding style predominant in that area. Over the past few years our petrological studies from a widespread lateral and vertical sampling of the cross-bedded Coconino shows that its petrology is inconsistent with the expectations for eolian depositional environments. We have found dolomite ooids, beds, cement, and clasts at a number of widespread places within the formation. Our petrographic thin sections show the sand is not well-sorted or well-rounded (the same results were also obtained by Lundy [1973]). Additionally, we have found muscovite flakes and angular K-feldspar sand in most of the >250 thin sections we have examined from the Coconino. It is hard to explain how muscovite could survive abrasive eolian conditions and how K-feldspar grains could remain so angular in such an environment. Blakey (1984) thought that at least part of the Schnebly Hill Formation was deposited by marine sand waves. It has long been recognized that much of the Toroweap is marine (Blakey and Knepp 1989; Rawson and Turner-Peterson 1980). We think that the identification of PRFs in putatively eolian Permian sandstones in Arizona provides convincing field evidence for the subaqueous origin of at least parts of these units.
Some may suggest that the PRFs that we are describing represent fluvial deposits in an eolian sand sea or coastal dunes that have migrated into the ocean. We failed to find any evidence to support these hypotheses. The recumbently folded beds do not appear to be in any kind of channel that is laterally constrained. When folded beds are traced laterally, they transition into “normal” cross-bedding. On the other hand, we might expect radical changes in lithology and bedding types if these were fluvial deposits or seaward-migrating dunes. The transitional facies and sedimentary structures that would be expected are not present. There are planar-beds underlying and/or overlying the recumbent folds, but these would probably not be expected in a fluvial setting or transitional marine setting. We think Lundy’s (1973) hypothesis that the Coconino represents marine sand waves remains a viable hypothesis. Large sand waves are now known from over 40 locations around the world (Garner and Whitmore 2011). Most locations consist of large sand sheets on the continental shelf in areas with strong currents that would be capable of creating cross-beds and possibly PRFs. The internal geometry of sand waves has in a few cases been studied in seismic surveys and large-scale, steeply dipping cross-beds have been found (e.g., Berné, Auffret, and Walker 1988).
Others may suggest that the folds are due to large-scale dune collapse or slumping of wet (not subaqueous) material. However, folding that occurs by these mechanisms is small-scale and quite distinctive with associated faults (McKee and Bigarella 1979). The folds can be traced down-dip in a continuous bed for 170 m (557.7 ft) along Brins Ridge and for 50 m (164 ft) on Capitol Butte. It is hard to see how slumping can explain this. Another possibility might be slumping and folding due to seismic deformation, but those features are distinctive as well; such sediments contain small thrust faults, evidence of compaction, and convolute beds (Alsop and Marco 2011). We did see these kinds of features in one part of the Schnebly Hill Formation (Fig. 3, label B). Those beds could be traced uninterrupted for hundreds of meters and were observed at several locations throughout the Sedona area at the same stratigraphic level, making a seismic origin for them more feasible. Based on the available literature regarding PRFs and our observations from northeast Ohio, folds fade in and out of cross-bedded sands and are not present everywhere in a single bed; although they can be traced much further than the deformation associated with a slumped eolian dune face.
We are not sure why these features seem to be concentrated in the Sedona area. We have visited many other Coconino outcrops, and Sedona seems to be the only place that has these types of deformation features in abundance. It may be that these features are more common than we think, but have so far been overlooked in the field. Even though PRFs are abundant in the Sharon Conglomerate of northeastern Ohio, the folds are much easier to see when the rock is jointed perpendicular to strike; otherwise they are difficult to find. Even in the Sharon Conglomerate the folds are discontinuous. In some places they are abundant, and in other places they are difficult to find. The Sedona area has hundreds of hiking trails that have been explored by one of our authors. Perhaps the frequency of folds in Sedona is a combination of the discontinuous nature of these folds and the large amount of rock exposure in Sedona. Another possibility is that water currents may have been stronger in the Sedona area. We have noticed that vertebrate tracks in the Coconino (described by Brand [1979] and Brand and Tang [1991]) are much more common in areas like the Grand Canyon (where we have found no examples of these folds) and virtually absent in the Sedona area (where the folds are quite common). Planar-beds (which are often associated with the folds) are common in the Sedona area, and much less common in the Grand Canyon area. This may suggest strong currents and changing flow regimes in the Sedona area.
Convoluted beds are quite common in cross-bedded sandstones of purported eolian origin (e.g., Bryant and Miall 2010; Doe and Dott 1980). Many of these deformation features are not of the type we are describing within this paper (Types I and II). However, based on photographs and drawings contained within Knight (1929) and Steidtmann (1974) the depositional environment of the Casper Formation of Wyoming might be worth reconsidering because it appears to contain PRFs. Knight originally identified the formation as subaqueous, but it seems the current consensus is eolian (McKee 1979). Based on one small example of a parabolic recumbent fold in our precursory examination of the Tensleep Sandstone, also in Wyoming (Fig. 16), it might be worth searching for these features more widely in the Tensleep. The Tensleep is another formation that has had both aqueous and eolian interpretations of its cross-beds (e.g., Kerr and Dott 1988). We encourage reexamination of the deformation in all cross-bedded sandstones to see whether they contain PRFs or not. It appears these types of folds are an overlooked but important indicator of subaqueous depositional environments in ancient cross-bedded sandstones.
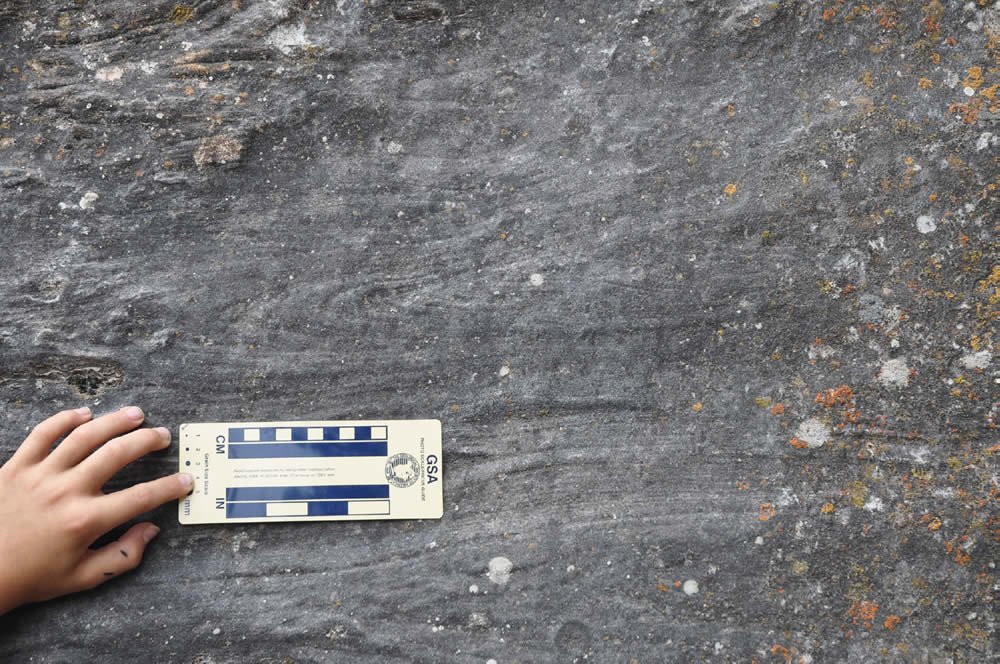
Fig. 16. A small parabolic recumbent fold in the Tensleep Formation, Ten Sleep Canyon, Wyoming.
At this point we should consider the words of William Morris Davis (1926, p. 464): “But to make such progress [in geology and physics], violence must be done to many of our accepted principles; and it is here that the value of outrageous hypotheses, of which I wish to speak, appears. For inasmuch as the great advances of physics in recent years and as the great advances of geology in the past have been made by outraging in one way or another a body of preconceived opinions, we may be pretty sure that the advances yet to be made in geology will be at first regarded as outrages upon the accumulated convictions of to-day, which we are too prone to regard as geologically sacred.”
Conclusion
We have shown that intraformational parabolic recumbent folds (PRFs) are present in the cross-bedded portions of the Schnebly Hill, Coconino Sandstone, and Toroweap Formations in the area around Sedona, Arizona. These types of structures can only be formed by strong water currents in a subaqueous setting. We base our conclusions on the following lines of evidence: 1) These kinds of structures have only been reported from subaqueous depositional settings (both fluvial and marine). 2) These kinds of structures have been formed in laboratory settings, but only subaqueously. 3) These kinds of structures have not been found in modern eolian settings. 4) Deformational structures known in modern eolian dunes are quite different in form and scale from those we report. 5) Our petrographic observations are more consistent with a subaqueous than an eolian environment for the Coconino. Several mechanisms are available to cause syndepositional liquefaction and deformation of cross-beds. In this case, we believe that the liquefaction was caused either by changes in flow regime or cyclic loading of the water column above the cross-beds during deposition. We suggest that a subaqueous sand wave hypothesis be reconsidered for parts of the Schnebly Hill, Coconino Sandstone and Toroweap Formations.
Acknowledgments
We wish to acknowledge Cedarville University, Calgary Rock and Materials Services Inc., Institute for Creation Research, and some other private donors for financial and logistical help. Without this assistance, this report would have been impossible. We thank various reviewers who have helped us greatly improve the manuscript.
References
Allen, J. R. L., and N. L. Banks. 1972. An interpretation and analysis of recumbent-folded deformed cross-bedding. Sedimentology 19, no. 3–4:257–283.
Alsop, G. I., and S. Marco. 2011. Soft-sediment deformation within seismogenic slumps of the Dead Sea Basin. Journal of Structural Geology 33, no. 4:433–457.
Berné, S., J.-P. Auffret, and P. Walker. 1988. Internal structure of subtidal sandwaves revealed by high-resolution seismic reflection. Sedimentology 35, no. 1:5–20.
Bhattacharya, H. N., and S. Bandyopadhyay. 1998. Seismites in a Proterozoic tidal succession, Singhbhum, Bihar, India. Sedimentary Geology 119, no. 3–4:239–252.
Billingsley, G. H., S. S. Priest, and T. J. Felger. 2007. Geologic map of Wupatki National Monument and vicinity, Coconino County, northern Arizona. US Geological Survey, Scientific Investigations Map 2958.
Blakey, R. C. 1984. Marine sand-wave complex in the Permian of central Arizona. Journal of Sedimentary Petrology 54, no. 1:29–51.
Blakey, R. C., and R. Knepp. 1989. Pennsylvanian and Permian Geology of Arizona. Arizona Geological Society Digest 17:313–347.
Brand, L. R. 1979. Field and laboratory studies on the Coconino Sandstone (Permian) vertebrate footprints and their paleoecological implications. Palaeogeography, Palaeoclimatology, Palaeoecology 28:25–38.
Brand, L. R. 1992. Fossil vertebrate footprints in the Coconino Sandstone (Permian) of northern Arizona: Evidence for underwater origin: Reply. Geology 20, no. 7:668–669.
Brand, L. R., and T. Tang. 1991. Fossil vertebrate footprints in the Coconino Sandstone (Permian) of northern Arizona: Evidence for underwater origin. Geology 19, no. 12:1201–1204.
Bryant, G., and A. Miall. 2010. Diverse products of near-surface sediment mobilization in an ancient eolianite: Outcrop features of the early Jurassic Navajo Sandstone. Basin Research 22, no. 4:578–590.
Coleman, J. M. 1969. Brahmaputra River: Channel processes and sedimentation. Sedimentary Geology 3, no. 2–3:129–239.
Coleman, J. M., and S. M. Gagliano. 1965. Sedimentary structures: Mississippi River deltaic plain. In Primary sedimentary structures and their hydrodynamic interpretation, ed. G. V. Middleton, 133–148. Tulsa, Oklahoma: SEPM Special Publication 12.
Cosentino, A. J. 2007. Recumbently deformed crossbeds in the Spoon Formation sandstone, eastern Iowa. Compass 80, no. 2:45–53.
Davis, W. M. 1926. The value of the outrageous geological hypothesis. Science 63, no. 1636:463–468.
Doe, T. W., and R. H. Dott, Jr. 1980. Genetic significance of deformed crossbedding; with examples from the Navajo and Weber Sandstones of Utah. Journal of Sedimentary Petrology 50, no. 3:793–812.
Dott, R. H. 1966. Eocene deltaic sedimentation at Coos Bay, Oregon. Journal of Geology 74, no. 4:373–420.
Emery, M. K., S. A. Maithel, and J. H. Whitmore. 2011. Can compaction account for lower-than-expected cross-bed dips in the Coconino Sandstone (Permian), Arizona? Geological Society of America Abstracts with Programs 43, no. 5:430.
Fisher, W. L. 1961. Upper Paleozoic and Lower Mesozoic stratigraphy of Parashant and Andrus Canyons, Mohave County, Northwestern Arizona. PhD dissertation. Lawrence, Kansas: University of Kansas.
Foos, A. M., ed. 2003. Pennsylvanian Sharon Formation, past and present: Sedimentology, hydrogeology, and historical and environmental significance. Columbus, Ohio: State of Ohio, Department of Natural Resources, Division of Geological Survey, Guidebook no. 18.
Garner, P. A., and J. H. Whitmore. 2011. What do we know about marine sand waves? A review of their occurrence, morphology and structure. Geological Society of America Abstracts with Programs 43, no. 5:596.
Greb, S. F., and A. W. Archer. 2007. Soft-sediment deformation produced by tides in a meizoseismic area, Turnagain Arm, Alaska. Geology 35, no. 5:435–438.
Hendry, H. E., and M. R. Stauffer. 1975. Penecontemporaneous recumbent folds in trough cross-bedding of Pleistocene sands in Saskatchewan, Canada. Journal of Sedimentary Petrology 45, no. 4:932–943.
Horowitz, D. H. 1982. Geometry and origin of large-scale deformation structures in some ancient wind-blown sand deposits. Sedimentology 29, no. 2:155–180.
Hurst, A., and K. W. Glennie. 2008. Mass-wasting of ancient aeolian dunes and sand fluidization during a period of global warming and inferred brief high precipitation: The Hopeman Sandstone (late Permian), Scotland. Terra Nova 20, no. 4:274–279.
Jones, G. P. 1962. Deformed cross-stratification in Cretaceous Bima Sandstone, Nigeria. Journal of Sedimentary Petrology 32, no. 2:231–239.
Kerr, D. R., and R. H. Dott Jr. 1988. Eolian dune types preserved in the Tensleep Sandstone (Pennsylvanian-Permian), north-central Wyoming. Sedimentary Geology 56, no. 1–4:383–402.
Knight, S. H. 1929. The Fountain and the Casper Formations of the Laramie Basin. University of Wyoming Publications in Science: Geology 1, no. 1:1–82.
Lockley, M. G. 1992. Fossil vertebrate footprints in the Coconino Sandstone (Permian) of northern Arizona; evidence for underwater origin: Comment. Geology 20, no. 2:666–667.
Lundy, W. L. 1973. The stratigraphy and evolution of the Coconino Sandstone of northern Arizona. MS thesis. Tulsa, Oklahoma: University of Tulsa.
Mazumder, R., and W. Altermann. 2007. Discussion on new aspects of deformed cross-strata in fluvial sandstones: Examples from Neoproterozoic formations in northern Norway by S. L. Røe and M. Hermansen. Sedimentary Geology 198, no. 3–4:351–353.
McCormick, C. D., and M. D. Picard. 1969. Petrology of Gartra Formation (Triassic), Uinta Mountain area, Utah and Colorado. Journal of Sedimentary Petrology 39, no. 4: 1484–1508.
McKee, E. D. 1934. The Coconino Sandstone—its history and origin. In Papers concerning the palaeontology of California, Arizona, and Idaho, 77–115. Washington D.C.: Carnegie Institution Publication No. 440.
McKee, E. D. 1938. Original structures in Colorado River flood deposits of Grand Canyon. Journal of Sedimentary Petrology 8, no. 3:77–83.
McKee, E. D. 1962. Origin of the Nubian and similar sandstones. Geologische Rundschau 52:551–587.
McKee, E. D. 1979. Ancient sandstones considered to be eolian. In A study of global sand seas, ed. E. D. McKee, 187–238. US Geological Survey Professional Paper 1052.
McKee, E. D., and J. J. Bigarella. 1979. Sedimentary structures in dunes. In A study of global sand seas, ed. E. D. McKee, 83–134. US Geological Survey Professional Paper 1052.
McKee, E. D., J. R. Douglass, and S. Rittenhouse. 1971. Deformation of lee-side laminae in eolian dunes. Geological Society of America Bulletin 82, no. 2:359–378.
McKee, E. D., M. A. Reynolds, and C. H. Baker. 1962a. Experiments on intraformational recumbent folds in crossbedded sand. US Geological Survey Professional Paper 450-D:155–160.
McKee, E. D., M. A. Reynolds, and C. H. Baker. 1962b. Laboratory studies on deformation in unconsolidated sediment. US Geological Survey Professional Paper 450- D:151–155.
Molina, J. M., P. Alfaro, M. Moretti, and J. M. Soria. 1998. Soft-sediment deformation structures induced by cyclic stress of storm waves in tempestites (Miocene, Guadalquivir Basin, Spain). Terra Nova 10, no. 3:145–150.
Owen, G. 1996. Experimental soft-sediment deformation: Structures formed by the liquefaction of unconsolidated sands and some ancient examples. Sedimentology 43, no. 2:279–293.
Owen, G., and M. Moretti. 2011. Identifying triggers for liquefaction-induced soft-sediment deformation in sands. Sedimentary Geology 235, no. 3–4:141–147.
Owen, G., M. Moretti, and P. Alfaro. 2011. Recognising triggers for soft-sediment deformation: Current understanding and future directions. Sedimentary Geology 235, no. 3–4: 133–140.
Peirce, H. W., N. J. Jones, and R. Rogers. 1977. A survey of uranium favorability of Paleozoic rocks in the Mogollon Rim and slope region—east central Arizona. Tucson, Arizona: State of Arizona Bureau of Geology and Mineral Technology, Geological Survey Branch, Circular 19.
Rawson, R. R., and C. E. Turner-Peterson. 1980. Paleogeography of northern Arizona during the deposition of the Permian Toroweap Formation. In Paleozoic Paleogeography of West- Central United States Rocky Mountain Paleogeography Symposium 1, ed. T. D. Fouch and E. R. Magathan, 341– 352. Denver, Colorado: Rocky Mountain Section, SEPM.
Reineck, H.-E., and I. B. Singh. 1980. Depositional sedimentary environments: With reference to terrigenous clastics. New York, New York: Springer-Verlag.
Rettger, R. E. 1935. Experiments on soft-rock deformation. Bulletin of the American Association of Petroleum Geologists 19, no. 2:271–292.
Robson, D. A. 1956. A sedimentary study of the Fell Sandstones of the Coquet Valley, Northumberland. Quarterly Journal of the Geological Society London 112:241–262.
Røe, S.-L., and M. Hermansen. 2006. New aspects of deformed cross-strata in fluvial sandstones: Examples from Neoproterozoic formations in northern Norway. Sedimentary Geology 186, no. 3–4:283–293.
Rust, B. R. 1968. Deformed cross-bedding in Tertiary- Cretaceous sandstone, Arctic Canada. Journal of Sedimentary Petrology 38, no. 1:87–91.
Samaila, N. K., M. B. Abubakar, E. F. C. Dike, and N. G. Obaje. 2006. Description of soft-sediment deformation structures in the Cretaceous Bima Sandstone from the Yola Arm, Upper Benue Trough, northeastern Nigeria. Journal of African Earth Sciences 44, no. 1:66–74.
Steidtmann, J. R. 1974. Evidence for eolian origin of cross-stratification in sandstone of the Casper Formation, southernmost Laramie Basin, Wyoming. Geological Society of America Bulletin 85, no. 12:1835–1842.
Stewart, J. H. 1961. Origin of cross-strata in fluvial sandstone layers in the Chinle Formation (Upper Triassic) on the Colorado Plateau. US Geological Survey Professional Paper 424-B:127–129.
Visher, G. S. 1990. Exploration stratigraphy. 2nd ed. Tulsa, Oklahoma: PennWell Publishing Co.
Wells, N. A., S. S. Richards, S. Peng, S. E. Keattch, J. A. Hudson, and C. J. Copsey. 1993. Fluvial processes and recumbently folded crossbeds in the Pennsylvanian Sharon Conglomerate in Summit County, Ohio, USA. Sedimentary Geology 85, no. 1–4:63–83.
Whitmore, J. H., G. Forsythe, R. Strom, and P. A. Garner. 2011. Unusual bedding styles for the Coconino Sandstone (Permian), Arizona. Geological Society of America Abstracts with Programs 43, no. 5:433.
Whitmore, J. H., R. Strom, S. Cheung, and P. A. Garner. 2014. The Petrology of the Coconino Sandstone (Permian), Arizona, USA. Answers Research Journal 7:499–532. https://answersingenesis.org/geology/rock-layers/petrology-of-the-coconino-sandstone/
Yagishita, K., and R. C. Morris. 1979. Microfabrics of a recumbent fold in cross-bedded sandstones. Geological Magazine 116, no. 2:105–116.