Research conducted by Answers in Genesis staff scientists or sponsored by Answers in Genesis is funded solely by supporters’ donations.
Abstract
The sedimentary strata that cover most of Israel are an obvious record of the Genesis Flood. A major erosion surface (unconformity) at the base of the sedimentary sequence cut across the Precambrian (pre-Flood) crystalline basement rocks. This resulted from the catastrophic passage of the Flood waters as they rose in enormous tsunami-like surges over the continental land at the initiation of the Flood event. These rising Flood waters transported sediments and marine organisms over the continental land. Many thousands of meters of marine sediments were thus deposited on a vast scale across Israel, rapidly burying myriads of marine organisms in fossil graveyards. Land organisms were similarly overwhelmed by the Flood waters, their remains buried with the marine organisms. The global extent of some of these sedimentary layers in Israel is confirmed by correlations of strata across and between continents, such as the sandstone with pebbles at the base of the Flood sequence, and the massive pure chalk beds at the top. The biblical account of the Flood describes the formation of mountains from halfway through to the end of the year-long Flood event. Thus late in the Flood powerful tectonic upheaval processes overturned and upthrust Flood-deposited sedimentary strata to form these mountains. Simultaneous isostatic adjustments also resulted in restoring continental land surfaces as the Flood waters receded and drained into new deep ocean basins. In Israel this great regression is marked by the end of the widespread “marine” sedimentation and an erosion surface across the country. The subsequent minor local continental sedimentation represents residual post-Flood geologic activity. The end of the Flood also coincided with the commencement of the rifting that opened the Red Sea and the Dead Sea-Jordan River rift valley, as well as the uplifting of the Judean Mountains and the upthrusting of Mt. Hermon.
Keywords: Israel, geology, Flood, sedimentary strata, fossils, erosional unconformities, e-Flood/Flood boundary, Flood/post-Flood boundary
Introduction
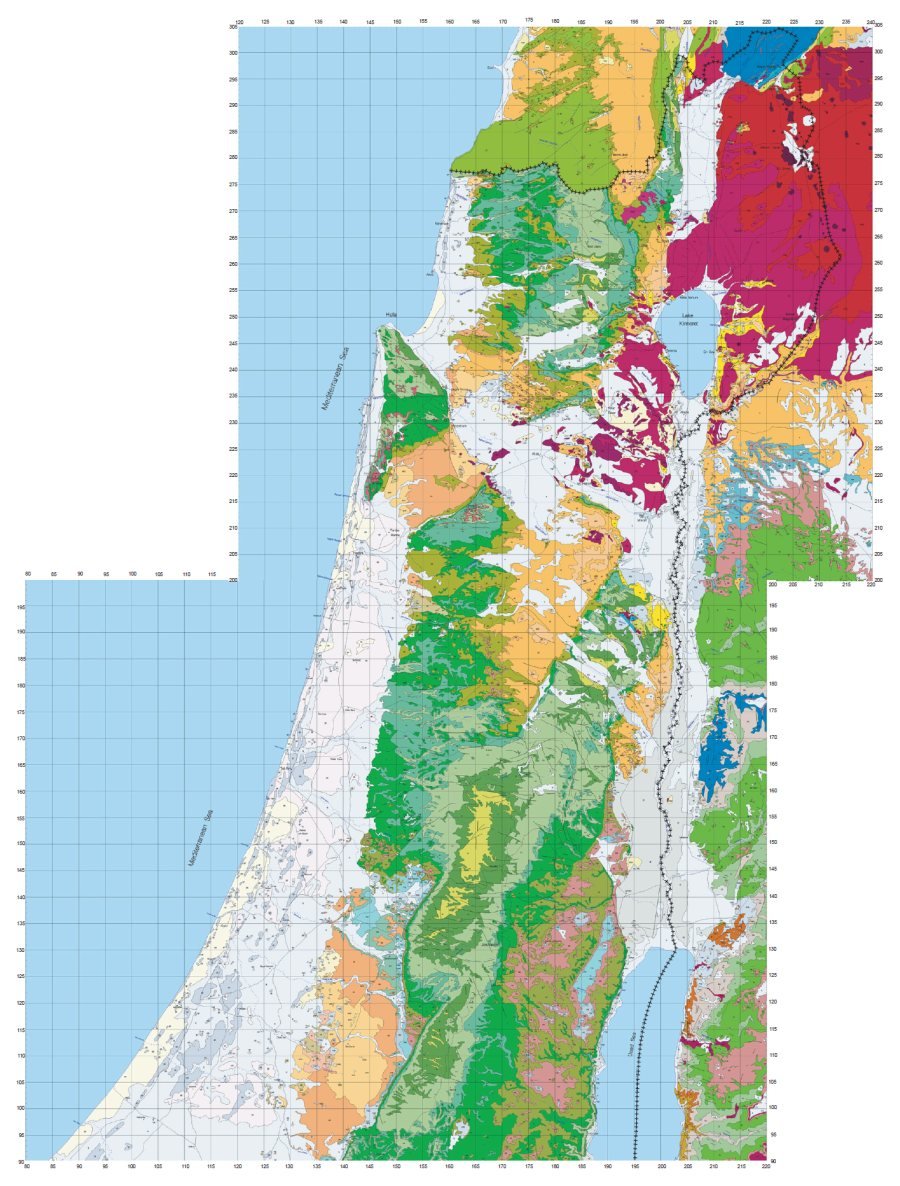
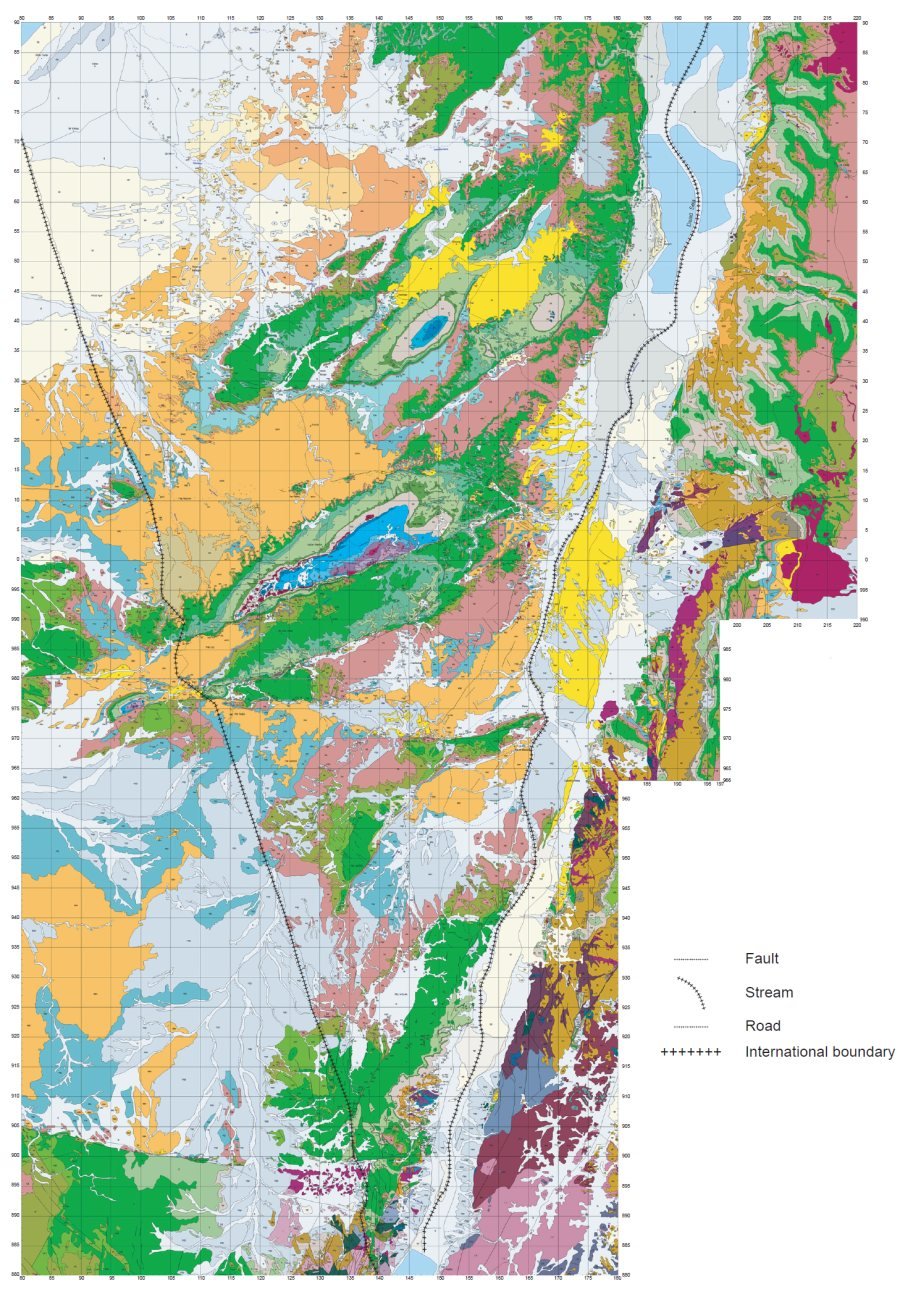
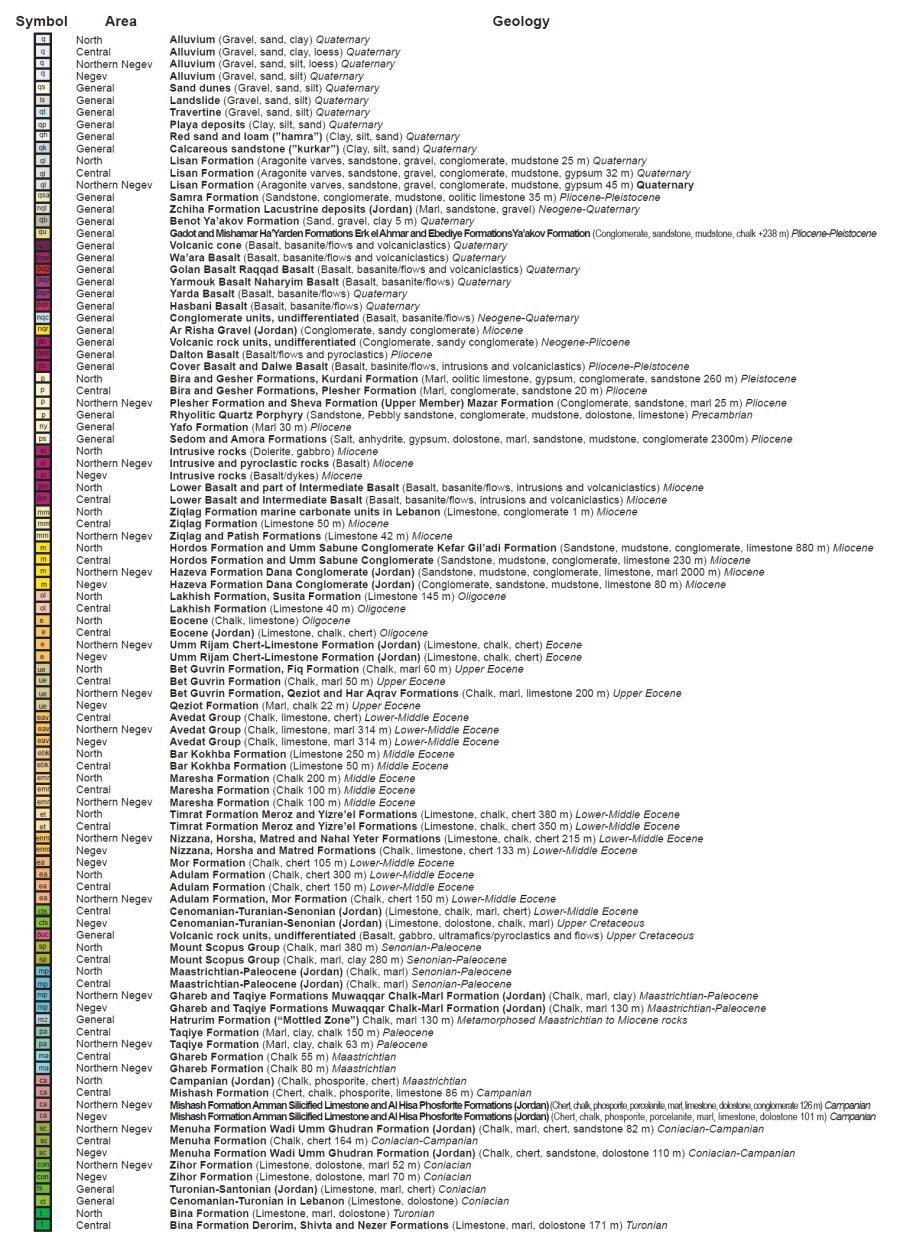
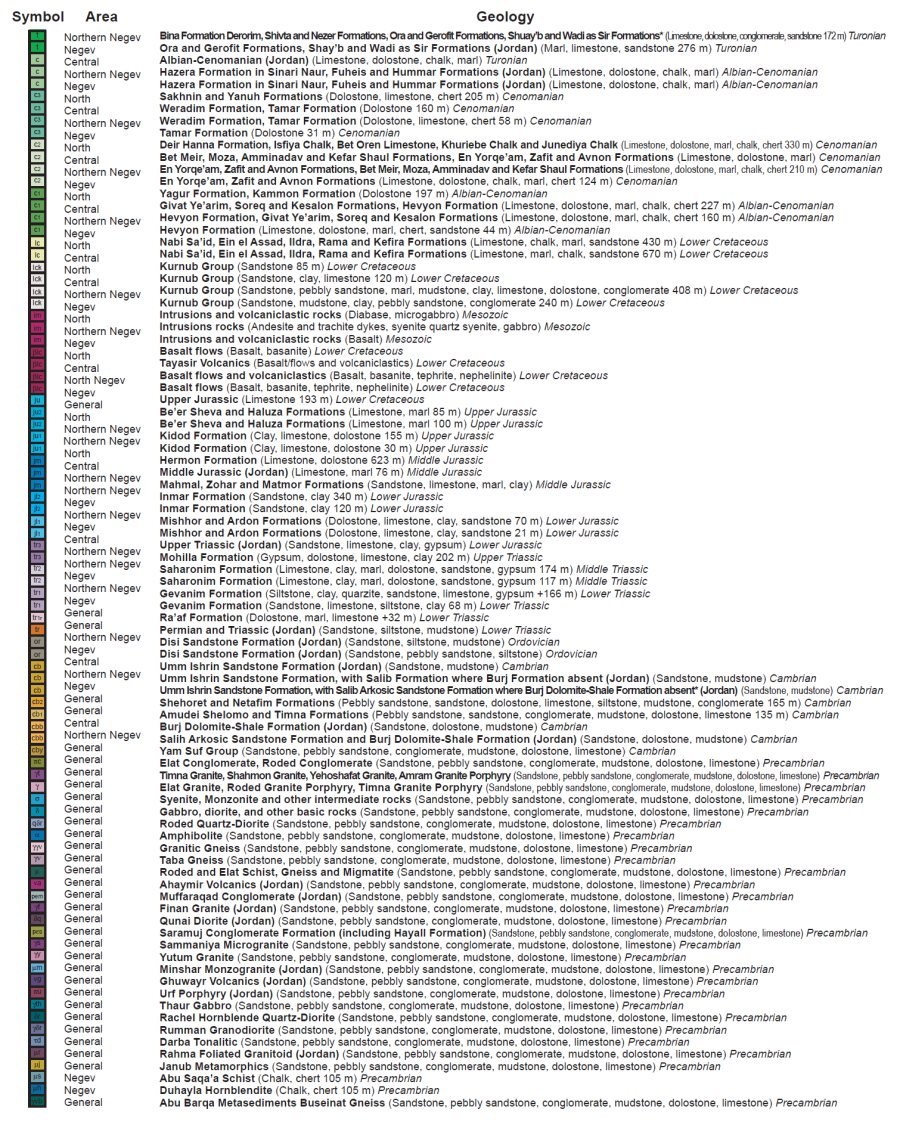
Fig. 1 (pp. 268–271). Detailed geologic map of Israel, in two adjoining sheets, from the mountains of Lebanon in the north (northern sheet) to the Negev and Red Sea in the south (southern sheet) (after Sneh et al. 1998). The only Precambrian (pre-Flood) rocks are found in the far south of the country near Elat. Otherwise most of Israel is covered by Flood rocks. Details of most of the rock units on the map are listed in the legend.
As the land of the Bible, the geology of Israel is the setting for the unfolding post-Flood account of God’s plan for our salvation. When Jesus “went up to Jerusalem” from the region of Galilee to go to Calvary for us, he had to climb through hills of fossiliferous limestone and chalk deposited by God’s Flood judgment of man’s sin (Snelling 2009a). Thus understanding the geology of Israel from a biblical perspective of earth history adds to our appreciation of what we read in the Scriptures. Furthermore, because some post-Flood events recorded in the Scriptures likely affected the geology of Israel, identifying those effects may aid our alignment of the global geologic record within the biblical framework of history.
The year-long global catastrophic Flood of Genesis 6–9 is the event which divides the global geologic record into its three main sections—pre-Flood, Flood, and post-Flood rocks. Snelling (2010a) identified and discussed the pre-Flood rocks of Israel, found only in the Elat area in the far south of the country. The unconformity across the top of the Precambrian igneous and metamorphic basement rocks was suggested as marking the onset of the Flood, which also included the rapid deposition of coarse clastic sediments (arkose and arkosic conglomerate) accompanied by volcanics (basalt flows, some erupted under water, and explosively erupted tuffs and other pryoclastics) (Garfunkel 1980), consistent with the breaking up of the pre-Flood crust as waters of the fountains of the great deep erupted.
The initiation of this breaking up of the pre-Flood crust triggered the catastrophic plate tectonics that provides a coherent, all embracing model for the Flood event and its contribution to the global geologic record (Austin et al. 1994; Baumgardner 2003). A fuller treatment of the application of that model to the geologic record within the biblical creation-Flood framework of earth history is provided by Snelling (2009b). That treatment also includes presentation and discussion of the details of the Flood event and the evidences of catastrophic deposition of the Flood sediments, all of which is relevant to the descriptive overview here of the Flood rocks of Israel.
Flood Rock Units
Much of Israel consists of exposed Flood-deposited fossiliferous sedimentary strata, from the far north of the country down through the central “spine” of the Judean Hills to the Negev in the south (Freund 1978; Garfunkel 1978). Fig. 1 (in two parts) is a detailed geologic map of the whole country (Sneh et al. 1998). Figs. 2 and 3 are two depictions of the stratigraphic succession of the rock units across Israel (Bartov and Arkin 1980; Freund 1977; Ilani, Flexer and Kronfeld 1987). Fig. 2 provides more details of the rock types, while Fig. 3 is more stylistic and includes subsurface information obtained in boreholes.
In southern Israel the flat-lying sedimentary strata (sandstone, limestone and shale) stacked above the unconformity representing the beginning of the Flood are about 1.6 km (1 mi.) thick, similar to the strata sequence exposed in the Grand Canyon (Austin 1994; Beus and Morales 2003). It was originally expected that this approximately 1.6 km (1 mi.) thick Cambrian through Jurassic sedimentary sequence in southern Israel would be persistent in thickness into central and northern Israel, being concealed there beneath the widespread cover of Cretaceous limestone and chalk. However, at Makhtesh Ramon in the central Negev, where over 915 m (3,000 ft) of Triassic, Jurassic and Cretaceous sedimentary strata are exposed, drilling penetrated another 2,000 m (6,560 ft) of sedimentary strata before reaching the unconformity with the pre-Flood crystalline basement (Austin 1998a). Furthermore, at Ramallah (only 24 km or 15 mi. north of Jerusalem) drilling and seismic refraction profiling indicate that there is about 7,000 m (22,960 ft) of sedimentary strata down to the same unconformity. Similarly, drilling on the coast near Gaza penetrated some 6,000 m (19,680 ft.) of sedimentary strata before reaching the basement granite. Therefore, there is more than a three-fold thickening of Flood-deposited sedimentary strata in the subsurface beneath central and northern Israel and northwestward into the Mediterranean Sea basin. And given that these sedimentary layers contain abundant marine fossils, the ocean waters clearly rose and prevailed during the Flood, covering the region.
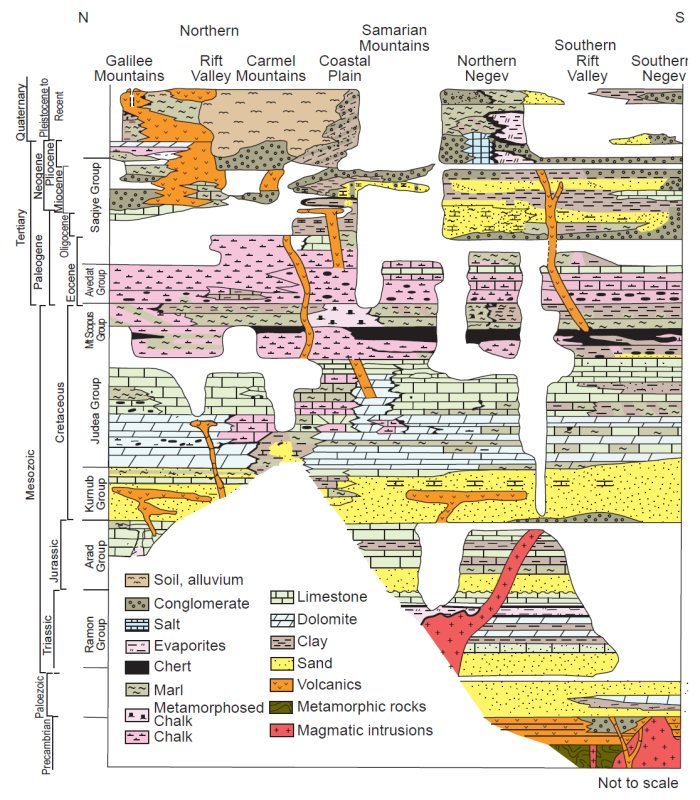
Fig. 2. A generalized stratigraphic chart showing the succession of rock units (their names and geologic ages) across Israel from south (right) to north (left) (after Barton and Arkin 1980; Ilani, Flexer and Kronfeld 1987).
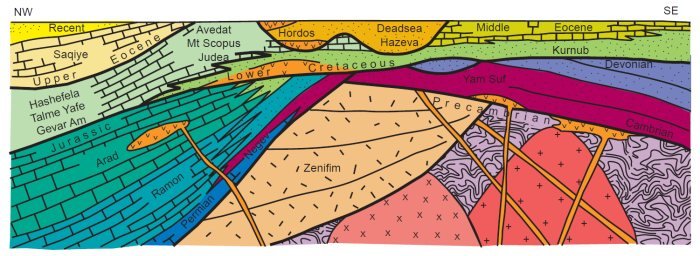
Fig. 3. The generalized stratigraphic sequence across Israel, extending from the Mediterranean coast in the northwest to Arabia in the southeast (after Freund 1977). The relationships between the major rock units of Israel’s geology are depicted, with heavy lines representing the regional unconformities separating six major straigraphic “packages” of strata. The conventional ages of the tops and bottoms of these “megasequences” are designated. Dotted areas indicate clastic rocks; bricks indicate shales and non-clastic (mainly calcareous) rocks; vs indicate volcanics.
Zenifim Formation (uppermost Neoproterozoic, terminal Precambrian)
As argued by Snelling (2010a), the first of the Flood rock units was likely the sediments and volcanics of the Zenifim Formation (fig. 3). In the Elat area polymictic conglomerates and volcanics outcrop in several small grabens (Bentor 1961; Garfunkel 1980). They lie on the eroded surface of the metamorphic and granitic basement rocks. The conglomerates contain clasts of all those older crystalline rocks, including the dikes. The matrix of the conglomerates is rich in lithic fragments and mafic minerals. Both clasts and matrix of these conglomerates are derived locally, indicating catastrophic erosion and nearby burial before fragile and weathering-prone minerals could disintegrate. These coarse conglomerates are interbedded with basalt and spilite flows (the latter indicating eruption under water), intermediate-acid volcanics, tuffs and pyroclastics (indicative of violent eruptions).
Grouped together informally as the Elat conglomerate, these conglomerates with interbedded volcanics have been correlated with the very similar Saramuj Conglomerate in Jordan, southwest of the Dead Sea and in Israel west of the Avara Valley, and with the Zenifim Formation, known only from boreholes in the Negev. Indeed, the outcropping Elat and Saramuj conglomerates are regarded as representing the margins of a large subsurface basin in which the Zenifim Formation, more than 2,800 m (9,180 ft) thick in the Ramon-1 well, accumulated (Garfunkel 1978; Wiessbrod 1969). This formation consists of arkose, similar to the matrix in the exposed conglomerates, and small amounts of finer clastics as well as volcanics.
While it has been argued (Snelling 2010a) that these conglomerates and the Zenifim Formation arkose and volcanics bear some resemblance positionally to the terminal Neoproterozoic Sixtymile Formation in the Grand Canyon and the Kingston Peak Formation and overlying units in the Mojave Desert (California) (Austin and Wise 1994) as the initial Flood deposits, a closer correlative may be the Mount Currie Conglomerate and Uluru Arkose of central Australia (Snelling 1998; Sweet and Crick 1992; Wells et al. 1970). The Mount Currie Conglomerate is also a coarse polymitic conglomerate with an arkose matrix identical to this unit’s lateral equivalent, the Uluru Arkose, which together are up to 6,000 m (19,680 ft) thick. These were once interpreted as glacial deposits (Holmes 1965). Similarly, Garfunkel (1980) describes the Elat conglomerate as sometimes “not unlike glacial deposits”. Instead, all these named and other rock units are excellent examples of the results of catastrophic submarine debris avalanches when the edges of the pre-Flood supercontinent collapsed as the break-up of the fountains of the great deep triggered the initiation of catastrophic plate tectonics (Austin et al. 1994; Austin and Wise 1994; Sigler and Wingerden 1998; Wingerden 2003). Furthermore, both the Zenifim Formation arkose and conglomerates in Israel, and the Mount Currie Conglomerate and Uluru Arkose in central Australia (Snelling 1998), are added testimony to the catastrophic erosion and deposition at the onset of the Flood cataclysm responsible for the rapid local accumulation of such enormous thicknesses of the immature sediments that were immediately buried by ongoing Flood sedimentation. The underwater and explosively erupted volcanics interbedded with the Zenifim Formation arkose and conglomerates are also consistent with the breaking up of the pre-Flood crust explosively releasing lavas as well as steam when the Flood began.
Yam-Suf Group (Cambrian–Devonian)
The exposed upper surface on the Precambrian metamorphic and granitic basement in southern Israel is a regular peneplain that extends over hundreds of square kilometers (Garfunkel 1978). In the Elat region the uppermost few meters of these basement rocks appear to have been deeply weathered before being covered by sediments. However, this weathering profile may have originally been up to hundreds of meters deep in the pre-Flood world, so that what remains is just a remnant after the severe deep erosion across this crystalline basement at the onset of the Flood. While the peneplain is usually a featureless plain, there is some local relief, sometimes quite rugged, amounting to 100–150 m (328 ft–492 ft) in the Elat area (Karcz and Key 1966).
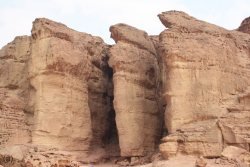
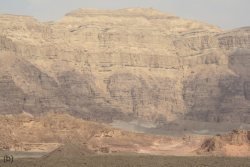
Fig. 4. The bedding in the sedimentary strata overlying the crystalline basement is parallel to the erosion surface across it. (a) The cliff-forming Amudei Shelomo Formation of the Cambrian Yam-Suf Group at Solomon’s Pillars, Timna. Note that the erosion surface (unconformity) across the crystalline basement is at the base of these cliffs. (b) The sedimentary strata sequence in the Timna area, with the Yam-Suf Group in the lower half of this cliff.
The overlying sedimentary strata are parallel to the surface of the basement (fig. 4). However, because their conventional age varies from place to place, from early Cambrian near Elat and south of the Dead Sea (the Amudei Shelomo Formation of the Yam-Suf Group) to late Carboniferous in the subsurface of the Negev, it is claimed that this peneplain was shaped during various periods in different places, supposedly over some 230 million years (Garfunkel 1978). Even its primary shaping during the early Cambrian in the Elat-Sinai region is suggested to possibly have taken 10–20 million years. But to suggest this vast flat peneplain was little modified over such a vast period is totally inconsistent with erosion processes and rates even in the present. It is far more reasonable for a single catastrophic erosive event to have swept across the region to shape the peneplain, such as the devastating tsunamis generated by the cataclysmic earthquakes as the pre-Flood crust broke up at the initiation of the Flood, that must have swept up and onto the pre-Flood land surface, deeply stripping weathered rock off it in a matter of hours to days. The different ages of the sediments then deposited onto that peneplained surface in different places would simply have been due to the successive sediment-laden tsunamis sweeping inland and depositing the various sediment layers in different places over the ensuing days of the Flood event.
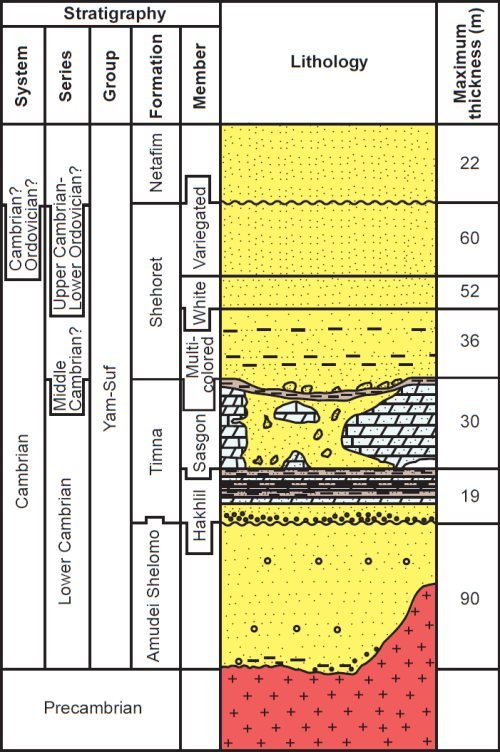
Fig. 5. Stratigraphic section of the Cambrian of southern Israel (after Segev 1984; Vermeesch, Avigad and McWilliams 2009). The deeply weathered and eroded upper Proterozoic granitic basement is unconformably overlain by lower Cambrian pebbly arkoses (sandstones) of the Amudei Shelomo Formation, subarkoses and carbonates of the Timna Formation, and fine-grained subarkoses and quartz-arenites of the Shehoret and Netafim Formations, all together making up the Yam-Suf Group.
The first rock units deposited on this exposed peneplain are those of the 300 m (984 ft) thick Cambrian–Lower Ordovician Yam-Suf Group, which is comprised of four formations (fig. 5). The first of these is the Amudei Shelomo Formation, which is up to 90 m (295 ft) thick and consists of brown, red and gray, relatively immature arkose to subarkosic sandstone, with fine- to grit-sized, rounded and poorly-sorted grains, with lenses or beds of quartzitic polymictic pebble conglomerate, often present at its base (Segev 1984; Vermeesch, Avigad and McWilliams 2009). Fig. 4a shows the full thickness of the flat-lying Amudei Shelemo Formation sandstone with its bedding paralleling the peneplained unconformity surface on top of the Precambrian crystalline basement, while Fig. 6 is a closer view of the unconformity at the same location. Fig. 7 shows the basal conglomerate at the unconformity, while Fig. 8 shows crossbedding within the arkosic sandstone. Both the basal conglomerate and the cross-bedding in the arkosic sandstone, together with the poorly-sorted mixture of mineral grains (especially feldspars) and rock clasts, are indicative of very rapid transport and deposition of this formation, consistent with the initial Flood conditions.
Unconformably overlying the Amudei Shelomo Formation is the Timna Formation, which consists of two members—the Hakhlil Member overlain by the Sasgon Member (fig. 5). The Hakhlil Member is in turn composed of four sub-units. At its base is a conglomerate comprising pink to brown polymictic, poorly-sorted, angular quartz porphyry fragments, which are up to 20 cm (8 in.) (in diameter (Segev 1984; Segev and Sass 1989). Overlying it are laminar red, fine-grained to coarse subarkosic sandstones (fig. 9). These are overlain by beds of fine-grained sandstone to grit cemented by calcite and dolomite, and sandy dolomite layers which alternate with red, purple and green siltstones and shales. The cemented sandstone beds exhibit cross-stratified internal structures and ripples. The uppermost subunit is composed of varicolored shales and siltstones containing thin beds and lenses of limestone and dolomite.
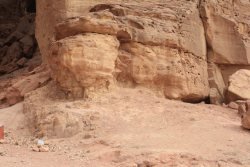
Fig. 6. The peneplained unconformity (erosion surface) on top of the Precambrian (pre-Flood) crystalline basement rocks beneath the Cambrian Amudei Shelomo Formation sandstone (arkose) at the base of the Flood sequence, Solomon’s Pillars, Timna.
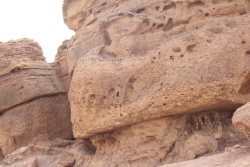
Fig. 7. The basal conglomerate in the Amudei Shelomo Formation, just above the unconformity with the Precambrian (pre-Flood) crystalline basement rocks, above Shehoret Canyon.
The upper part of the 45–50 m (147–164 ft) thick Timna Formation is the Sasgon Member, which is characterized by complicated lateral relationships between three distinctive lithofacies (fig. 5). These were originally defined as separate members, but the frequent and irregular transitions between them and the inability to map them has led to them being grouped together into the Sasgon Member. It is this member that hosts copper mineralization (Segev and Sass 1989), which was originally exploited by the Egyptians, and some uranium mineralization (Segev 1992). The first of these three lithofacies is a dolomitic lithofacies up to 28 m (92 ft) thick which is mainly composed of well-bedded brown to gray sandy dolomite with a few interbeds of shale, sandstone and limestone (Segev 1984, 1992; Segev and Sass 1989). Sedimentary structures include lamellar (rippled-form) stromatolites, gentle cross-stratification, ripple marks and trace fossils. Copper-rich horizons typify the base of this lithofacies (fig. 10). The main copper minerals in the dolomites are copper sulfides, but copper carbonate (malachite) also occurs in the sandstones (fig. 10). It has been proposed that intense weathering of the copper porphyry granites and quartz-porphyry dikes of the Timna Igneous Complex during the late Precambrian (late pre-Flood) provided the source of copper incorporated into these sediments (Shlomovitch, Bar-Matthews and Matthews 1999). The sandy lithofacies is distinguished by fine-grained to gritty subarkoses cemented by manganese and clay minerals. It is generally 5–7 m (16–23 ft) thick, reaching a maximum thickness of 21 m (69 ft). The upper part is laminar and contorted, the laminar structure reflecting regular alternation of variation in the content of black manganese oxides. The unit is commonly brecciated, mainly along intra-formational faults, with collapse structures, and copper and manganese mineralizations are dispersed throughout. The transitions between these two lithofacies is either abrupt, gradual or in the form of interfingering tongues (fig. 5). Blocks of the dolomitic lithofacies, in a wide range of sizes, are commonly found in the sandy lithofacies. The shaly lithofacies, which overlies both the dolomitic and sandy lithofacies, is usually only 2 m (6.6 ft) thick and is composed of light green, red or brown shales, siltstones and fine-grained subarkoses containing manganese and copper mineralizations.
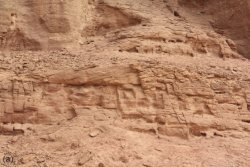
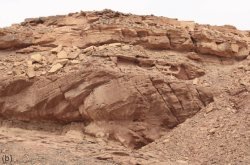
Fig. 8. Cross-bedding sets in the arkosic sandstone of the Amudei Shelomo Formation, which indicate rapid water transport of the sand (a) Solomon’s Pillars, Timna. (b) Above Shehoret Canyon.
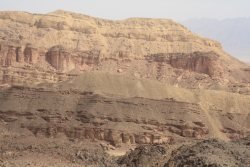
Fig. 9. The laminar red, fine-grained to coarse subarkosic sandstones of the Hakhlil Member of the Timna Formation can be seen just above halfway up this cliff near Shehoret Canyon.
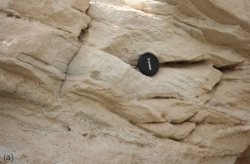
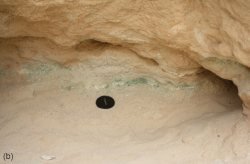
Fig. 10. Green malachite (copper carbonate) in the coarse sandstone of the Sasgon Member of the Timna Formation, within old mining tunnels first dug by the Egyptians in the Timna area. (a) Fine malachite grains following the laminations of cross-bedding in the sandstone. (b) Coarser malachite in a band within the sandstone.
Unconformably overlying the Timna Formation are the Shehoret and Netafim Formations, which together complete the Yam-Suf Group (fig. 5). The Shehoret Formation is up to 148 m (485 ft) thick and consists of fine- to coarse-grained subarkosic sandstones, which have been informally subdivided into a lower multi-colored unit, a middle white unit and an upper variegated unit (Segev 1984; Vermeesch, Avigad and McWilliams 2009). The 22 m (72 ft) thick Netafim Formation comprises fine-grained quartz arenite with alternating layers of siltstone and claystone. There is some disagreement over whether the Netafim Formation is upper Cambrian only, or transitional into the lower Ordovician.
The Cambrian designation of the Yam-Suf Group was established principally due to its basal position in the sedimentary strata sequence of Israel, where it sits directly and unconformably on the Precambrian crystalline basement. This is confirmed conventionally by the presence of both lower Cambrian brachiopods and trilobites found in the Timna Formation (Cooper 1976; Parnes 1971)—trilobites in both the Hakhlil Member and the sandy lithofacies of the Sasgon Member, and brachiopods in the dolomitic lithofacies of the Sasgon Member. These fossils would conventionally indicate that these rocks and the overlying formations are younger than 520 Ma (Landing et al. 1998).
Some age information has also been obtained from 40Ar/39Ar and U-Pb radioisotope dating of detrital K-feldspar and zircon grains respectively (Avigad et al. 2003; Kolodner et al. 2006; Vermeesch, Avigad and McWilliams 2009) (figs. 11 and 12). The detrital K-feldspar grains were obtained from a sample of the Shehoret Formation subarkosic sandstone, considered to have a depositional age of about 500 Ma. Fifty single-grain, K-feldspar, laser total-fusion extractions yielded a population of ages that tightly clustered around 535 Ma (lower Cambrian), indicating a single provenance and thermal history. About half of the grains yielded apparent ages that overlap with the very latest phase of igneous activity in the Precambrian basement, while all the grains are older than the depositional age. The 40Ar/39Ar age spectrum produced by step-heating yielded late Neoproterozoic to Cambrian apparent ages between 520 and 580 Ma, and a plateau age of about 560 Ma. However, none of these 40Ar/39Ar apparent ages is likely to represent the provenance age of these detrial K-felspar grains, as the oldest zircon U-Pb ages for suitable, close enough, source rocks are 580 Ma for an alkaline pluton intruding the Neoproterozoic Saramuj conglomerate (Jarrar, Wachendorf and Zellmer 1991; Jarrar, Wachendorf and Zachmann 1993), and 610 Ma for a Timna alkaline granite (Beyth et al. 1994).
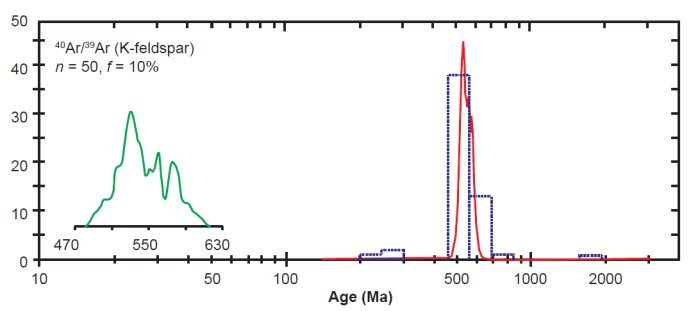
Fig. 11. Detrital grain-age distribution and density estimate of K-feldspar 40Ar/39Ar data (after Vermeesch, Avigad and McWilliams 2009). Note the logarithmic scale on the time axis of the main graph. A linear version of the hightemperature data is shown as an inset (left). Abbreviations: n = number of grains; f = smallest fraction sampled with >95% certainty.
The detrital zircon U-Pb ages (fig. 12) are more revealing. Avigad et al. (2003) extracted and analyzed detrial zircon grains from four sandstone samples, one from each of the four formations comprising the Yam-Suf Group, including one sample from the basal section of the Amudei Shelomo Formation. On the other hand, Kolodner et al. (2006) just focussed on the same sandstone sample Avigad et al. (2003) had collected from the Shehoret Formation. Nevertheless, the spread of detrital zircon U-Pb ages in the resultant histograms (fig. 12) was similar. The majority of grains yielded U-Pb ages less than 900 Ma, consistent with the conventional ages of the nearby underlying Neoproterozoic igneous and metamorphic basement rocks of the northern Arabian-Nubian Shield (Beyth et al. 1994; Halpern and Tristan 1981; Kröner, Eyal and Eyal 1990). However, there were also grains with Mesoproterozoic, Paleoproterozoic, and even Archean U-Pb ages, up to 3100 Ma. Indeed, the three groupings at 900–1100 Ma, 1650–1850 Ma, and 2450–2700 Ma represent about 30% of the total zircon grains analysed. These ages coincide with the crystalline basement rocks of the Saharan Metacraton of north Africa, the southeastern portion of the Arabian-Nubian Shield in Saudi Arabia, and granitoids in central Africa, which has led to the suggestion that some of these detrital zircon grains may have been transported up to 3,000 km (1,864 mi.) before deposition and burial in these Cambrian sandstones of southern Israel.
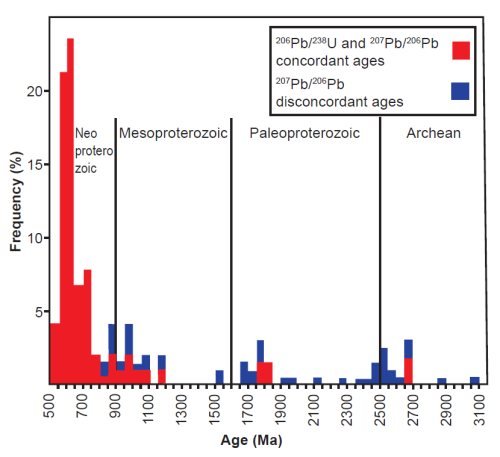
Fig. 12. Histogram showing age distribution of detrital zircons from the Cambrian siliciclastic section of southern Israel (after Avigad et al. 2003). Total number of zircons = 200. 157 grains yielded concordant ages. 206Pb/238U ages are used for zircons younger than 0.8 Ga; 207Pb/206Pb ages are quoted for older grains. 43 discordant grains are plotted on the basis of their 207Pb/206Pb ages.
Such an agreed long distance of sand transport by braided streams in littoral and shallow marine environments (Garfunkel 1978; Vermeesch, Avigad and McWilliams 2009) may be somewhat inconceivable, but during the onset of the global Flood cataclysm it is expected. Furthermore, the context of these sandstones is totally inconceivable unless their deposition was during the biblical Flood. Garfunkel (2002) describes the widespread distribution of early Paleozoic sediments right across north Africa to Arabia as “the largest sediment body preserved on earth” (Burke and Kraus 2000; Choubert and Faure-Mauret 1975; DeWitt et al. 1988). This 2,000 km (1,243 mi.) wide platform of far-traveled mature clastic sediments stretches from the west coast of north Africa to central Saudi Arabia, although only large “pockets” remain as a result of the subsequent erosion and reworking of those sediments. In southern Jordan and northwest Saudi Arabia this strata sequence thickens, and so extends up through the Ordovician and Silurian into the Devonian (Garfunkel 2002; Picard 1943; Weissbrod 1969). The same Cambrian–Silurian sedimentary layers also outcrop in both Syria and Turkey, and are easily recognized as “Nubian” sandstone in Egypt and Libya. Only relics remain as much of this vast and voluminous sediment body, comprising “the largest body of sediments ever deposited”, was eroded already before the Permian in Saudi Arabia and late Cretaceous in the Negev, with the detritus probably being swept as far south as the Karoo basins of southern Africa (Garfunkel 1978).
Such scales for a single vast and voluminous sediment body are not observed for any sediments being deposited today, nor such 3,000 km (1,864 mi.) long distances of sediment transport, to deposit, or to erode and carry away, such sediments. Yet these scales are to be expected in the global Flood cataclysm. Furthermore, a similar vast and voluminous body of sandstone, with a similar basal conglomerate, is found on another continent, and also sitting unconformably on a Precambrian crystalline basement. The Tapeats Sandstone in the Grand Canyon is the basal lithosome of the Sauk Megasquence, which covers, or once covered, much of North America (Austin 1994; Sloss 1963). As well as a basal conglomerate, with boulders up to 4.5 m (15 ft) wide, the base of the Tapeats Sandstone is often subarkosic, with K-feldspar grains ripped up from granites in the underlying Precambrian basement on which it sits unconformably (Austin 1994; Beus and Morales 2003). And Cambrian trilobites are found in the transition zone between the Tapeats Sandstone and the overlying, laterally deposited, Bright Angel Shale.
The similarity of the Amudei Shelomo Formation sandstone (figs 4, 6 and 7) to the Tapeats Sandstone is remarkable, given they now outcrop on different continents thousands of kilometers apart. Yet there is no question that they correlate as direct equivalents, both in their stratigraphic position and in their make-up. There is also the enormous scale of these continent-wide sand deposits, which were formed at the same time and in the same way. This is not to suggest they could have been the same single deposit of sand. Rather, they are consistent with a single global event forming them both at the same time in the same way. Nothing like this is happening today, so the present is not the key to the past, as conventionally thought. Today’s slow-and-gradual geologic processes are not depositing the same uniform sand beds with basal conglomerates on an unconformity surface right across two continents at the same time. These two very similar, equivalent and enormous sandstone layers are instead remarkable testimony to the onset of the global Flood cataclysm. With the breaking up of the pre-Flood crust, both oceanic and continental, and the initiation of catastrophic plate tectonics, the margin of the pre-Flood supercontinent collapsed, and the rising ocean waters energized into repeated tsunamis by the catastrophic earthquakes swept up onto and right across the continental plates, bringing sand and other sediments with them scraped off the shallow ocean floors, and eroded off the pre-Flood crystalline basement to produce more sand and other sediments, which were then deposited across that eroded and peneplaned, continent-wide unconformity surface (Austin et al. 1994; Austin and Wise 1994; Baumgardner 2003; Snelling 2009a).
Negev Group (upper Carboniferous–lower Triassic)
There is an erosive unconformity at the top of the upper Cambrian (-lower Ordovician?) Netafim Formation sandstone of the Yam-Suf Group in southern Israel (figs. 5 and 13). The equivalents of the Yam-Suf Group in southern Jordan and northwest Saudia Arabia are much thicker because they also include Ordovician, Silurian and lower Devonian sedimentary layers (fig. 13) (Garfunkel 2002; Picard 1943; Weissbrod 1969). And the same Cambrian– Silurian strata outcrop in Syria and Turkey, so it is likely that this whole thicker strata sequence was originally deposited right across Israel. Subsequently much of it was eroded from across Israel, leaving this truncated remnant in southern Israel, with just the erosive unconformity at the top as testimony to the enormous erosion that occurred. The scale of this erosion was continent-wide, with the detritus transported very long distances, for example, right across Africa to the south (Garfunkel 1978).
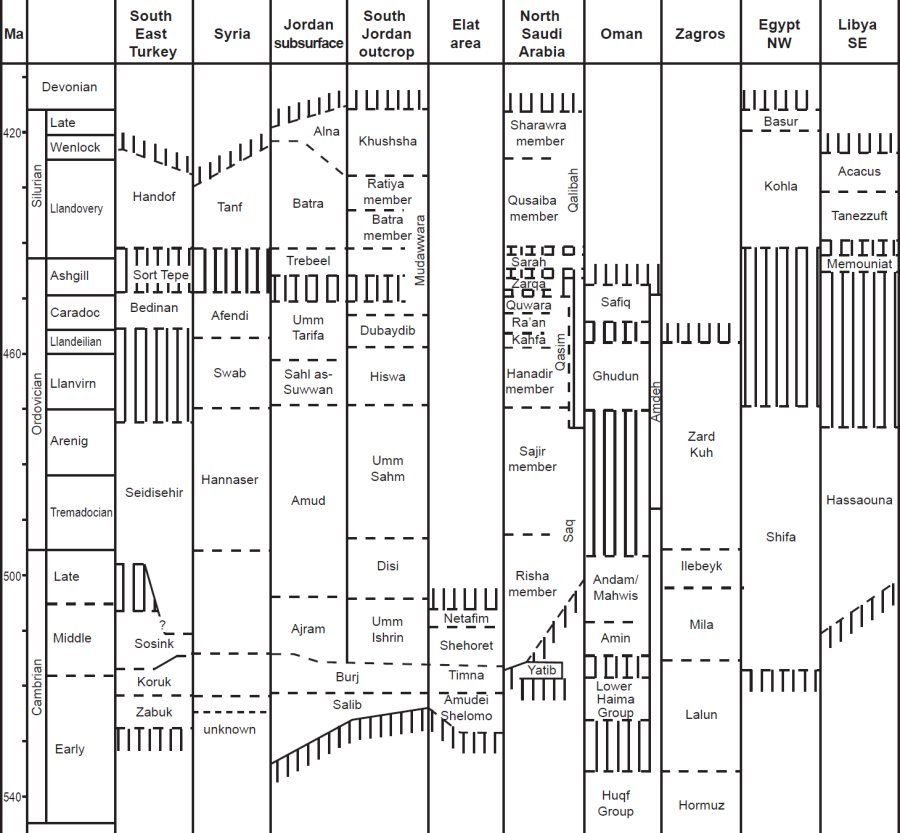
Fig. 13. Correlation chart of the Cambrian–Silurian stratigraphic units of Israel and surrounding countries (after Garfunkel 2002).
This again is only consistent with the scale of geologic processes during the Flood cataclysm. After the initial surges of the rising ocean waters across the continental plates, the water levels over the sediments on the continents would have dramatically fluctuated, due to the ebbs and surges caused by repeated tsunamis, and the tides which now resonated on a global ocean (Clark and Voss 1990; Snelling 2009b). Combined with rapid movements of the sediment-laden surfaces as the continental plates now moved at meters per second (Austin et al. 1994), any rapid continental-scale regression of the Flood waters would have catastrophically eroded into the previously deposited sediment layers on a massive scale, both in area and depth. Then with the next transgression as the Flood waters again surged across the continents, further erosion into the previously-deposited sediment layers would have occurred, followed close behind by the next cycle of rapid sedimentation. As this next “packet” of sediments was deposited, it would be inevitable that the layers deposited could involve lateral “facies” changes across the continents within the same megasequence, due to the mixture of sediment types in the surges, the water flow speeds, and how long the supply of the different sediment types lasted as they were water transported across the continents. Conventionally, these lateral “facies” have resulted in the different “facies” layers being given different formation names, when in fact such formations are lateral equivalents deposited at the same time from the same surges of Flood waters.
In southern Israel the Yam-Suf Group is overlain unconformably by quartzose sandstones of unknown age, though they are likely to still be Paleozoic (Weissbrod 1969). This is because the next cycle of sedimentation is known to have begun with upper Carboniferous sediments, based on sedimentary strata of upper Carboniferous and Permian conventional ages found in the subsurface of southern Israel, but also exposed around the northern part of the Gulf of Suez, in west central Sinai, and east of the Dead Sea (Garfunkel 1978; Wiessbrod 1969). In the subsurface of the Negev three formations have been defined:
- The Sa’ad Formation is essentially sandy, is upper Carboniferous, and lies unconformably on the terminal Precambrian (very earliest Flood) Zenifim Formation, or on volcanics.
- The Arqov Formation is upper Carboniferous-Permian and consists of alternating shales and carbonates, with few sandstones under the northern Negev, but becoming essentially sandy under the central Negev.
- The Yamin Formation is Permian, and consists mainly of carbonates, but sandstone is abundant in the south.
The total thickness of these sedimentary layers is 400–500 m (1,312–1,640 ft) (Garfunkel 1978; Weissbrod 1969). Together they have been grouped into the Negev Group (fig. 3). In the south they are truncated by the lower Carboniferous unconformity. Too little is known about these upper Carboniferous-Permian sedimentary layers in Israel and adjacent countries, but as their conventionally interpreted marine character becomes more pronounced to the north and northwest, it is presumed that the Permian transgression came from that direction. The Permian-Triassic boundary is not exposed, but probably occurs on top of the Yamin Formation. It is thus not clear whether there is a hiatus at that level. However, overlying the Yamin Formation, and exposed in Makhtesh Ramon in the central Negev, is the lower Triassic Zafir Formation, which consists mainly of shales with variable quantities of limestone. It has been also included in the Negev Group (Wiessbrod 1969). Its inclusion increases the total thickness of the sedimentary layers in this group to up to 600 m (1,968 ft) (Freund 1977).
Ramon Group (Triassic)
Triassic sedimentary rock units are well exposed in the central Negev, primarily in Makhtesh Ramon, a huge elongated crater-like erosional structure that has been called the “Grand Canyon” of Israel (Austin 1998a), where over 1,000 m (3,280 ft) of socalled Mesozoic strata are exposed (fig. 14). There are five Triassic named formations, the lowermost Zafir Formation (mainly shales and sandstones with variable quantities of limestone) being assigned to the Negev Group. The remaining four Triassic formations constitute the Ramon Group (Garfunkel 1978) (fig. 14):
- The Ra’af Formation consists mainly of limestones, with some dolomite, and siltstone and shale layers, with a rich marine fossil fauna. The rocks are mostly micrites and biomicrites.
- The Gevanim Formation is relatively rich in clastics—sandstones and siltstones in lower parts, and shales and siltstones in upper parts, which also contain fossiliferous limestones. The amount of shales and carbonates increases northward, in the subsurface.
- The Saharonim Formation consists mainly of carbonates, with lesser amounts of claystones and mudstones, and some sulfates (especially anhydrite and gypsum). The carbonates in the lower part are micrites, both biomicrites and grain-supported biomicrites. The amount of dolomite increases up the section, and so does the amount of sulfates. These are associated with fossil stromatolite beds and some flat pebble conglomerates. Concurrently the formation becomes less fossiliferous.
- The Mohilla Formation is characterized by a great development of anhydrite and gypsum (in exposures only) which are associated with dolomites and some shales. Oolites and beds with an impoverished fossil fauna are also present. This formation is characterized by abrupt facies changes, in contrast with the underlying formations in which facies changes are gradual.
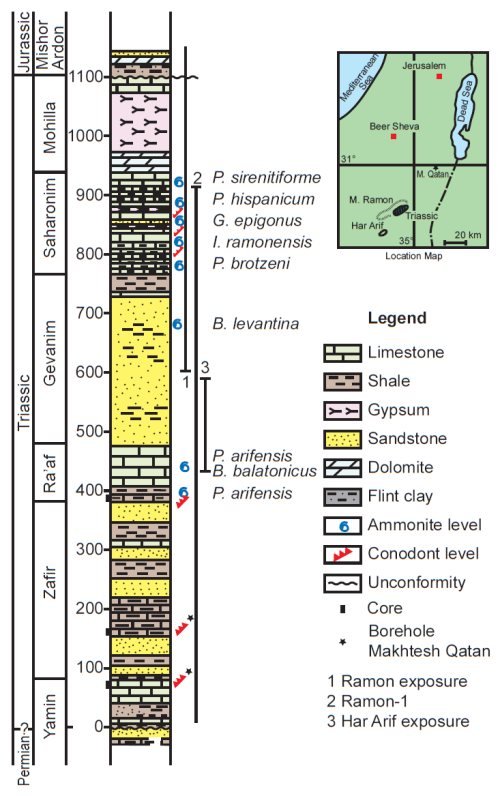
Fig. 14. Composite stratigraphic section of the Triassic sediment layers in southern Israel (after Parnes, Benjamini and Hirsch 1985). The locations from where exposed outcrops and boreholes were used to construct this composite stratigraphic section are shown in the inset location map.
Where well developed, the Triassic strata range in total thickness from 500 m (1,640 ft) to 1,100 m (3,608 ft). The Ra’af Formation is 70 m (230 ft) thick in the Ramon-1 borehole, but only 27 m (89 ft) of it are exposed at Har ‘Arif to the south of Makhtesh Ramon (fig. 14) (Parnes, Benjamini and Hirsch 1985). In Makhtesh Ramon the Gevanim Formation is 270 m (886 ft) thick (although only the upper 130 m (426 ft) are exposed), and the Saharonim Formation is 153–170 m (502–558 ft) thick (Benjamini, Druckman, and Zak 1993; Parnes, Benjamini and Hirsch 1985). The known thickness and facies variations of the Triassic formations are compatible with a pattern of NE–SW belts, and the distribution of the clastics, mainly sandstones, is compatible with a southeasterly provenance (Druckman 1974). However, a southwesterly provenance is equally probable, as paleocurrent measurements in the sandstones of the Gevanim Formation indicate the predominant direction of sediment transport was to the northeast (Karcz and Braun 1964; Karcz and Zak 1965, 1968). These paleocurrent measurements were derived from cross-beds that consistently dip at 15–25°, which is consistent with water transport of those sands (Austin 1994; Visher 1990).
The nature of the Ramon Group sediments themselves and their fossil contents (fig. 14) clearly indicate that ocean waters had flooded over the area, although the postulated depositional environments all involved only shallow waters (Garfunkel 1978). Carbonates are present in most of the Triassic sequence, with clastics (sandstones and shales) important in the lower part, and evaporites (precipitites) becoming common in the upper part (fig. 14). Open marine, shallow marine (subtidal, intertidal and supratidal), restricted (brackish to hypersaline), and continental depositional environments have all been postulated (Druckman 1974). Within the exposed stratigraphic section in Makhtesh Ramon, from the upper half of the Gevanim Formation through the Saharonim Formation to the Mohilla Formation, it is claimed there is evidence for some five coupled transgressive/regressive cycles (Benjamini, Druckman and Zak 1993), but these can be interpreted as representing oscillations in the Flood conditions.
Seven successive levels of ammonites are present in the Ramon Group, through the Ra’af, Gevanim and Saharonim Formations, which are useful for correlating these strata around the Mediterranean region (Parnes 1965; Parnes, Benjamini and Hirsch 1985). But these are not the only marine creatures fossilized in these rock units. The Saharonim Formation particularly has rich micro- and macrofossiliferous horizons, including the ammonites, with conodonts, bivalves, nautiloids, brachiopods, other molluscs, cephalopods, crinoids and echinoderms (Benjamini, Druckman and Zak 1993). Near the base of the formation is a limestone bed with a great many preserved cephalopods, with other nautiloids, and some ammonites. Sponges and corals are notably absent. Fossilized burrows are the main trace fossils, while foraminifers are the main microfauna. Algal structures are found in the limestone beds, and stromatolites increase in abundance upwards in the dolomite and evaporate (precipitite) beds through the Saharonim and Mohilla Formations. Some of these stromatolites are domal structures up to 2 m (6.6 ft) in diameter.
The Mohilla Formation is more than 200 m (656 ft) thick in Makhtesh Ramon, so this massive deposition of dolomite and gypsum/anhydrite evaporites (precipitites) warrants explanation. Rather than the conventional interpretation of a hypersaline environment in which these dolomites and sulfates slowly accumulated by evaporation, within the global Flood the catastrophic expulsion of hot saline hydrothermal fluids into the cold Flood waters can explain these deposits via rapid precipitation (Hovland et al. 2006; Snelling 2009b). Such hydrothermal fluids would have been associated with, and produced by, nearby magmatic and volcanic activity.
It is thus significant that also exposed in Makhtesh Ramon are a composite gabbro laccolith up to 90 m (295 ft) thick (Rophe, Eyal and Eyal 1993), basaltic and trachytic dikes and sills (Baer 1993), and stocks, bosses, dikes and sills of quartz syenite (Itmar and Baer 1993), all of which are indicative of prolonged and intense magmatic and volcanic activity in this region coinciding with the deposition of the sedimentary strata. The gabbro laccolith has been K-Ar dated at being emplaced between 136±4 Ma and 129±4 Ma (Lang et al. 1988), while the quartz syenite intrusions have been Rb-Sr dated at 107±12 Ma (Starinsky, Bielski and Steinitz 1980) and K-Ar dated at 130±5 Ma (Lang and Steinitz 1985). Such conventional early Cretaceous dates are consistent with these intrusions being younger than the sedimentary strata they intrude. The gabbro laccolith was emplaced between gypsum beds in the upper Triassic Mohilla Formation, and the quartz syenite intrusions are variously emplaced in the middle Triassic Gevanim Formation and Jurassic strata overlying the Ramon Group, while the basaltic and trachytic dikes and sills (also regarded as early Cretaceous) intruded into the Triassic Gevanim, Saharonim and Mohilla Formations and the overlying lower Jurassic units.
Conventionally, therefore, there could be no connection between this magmatic and volcanic activity and the deposition of the Mohilla Formation sulfate precipitites. On the other hand, however, within the year-long Genesis Flood there would have been only up to a few weeks between deposition of the Triassic strata and the lower Cretaceous emplacement of the intrusives. Thus the magma chambers that fed these intrusives had to already have been emplaced and active in the weeks preceding emplacement of the intrusives, so that the hot saline hydrothermal fluids associated with this magmatic activity could have been escaping along fractures into the Flood waters above to rapidly precipitate their dissolved salts to deposit the Mohilla Formation sulfates. Indeed, it is likely the intrusives were subsequently emplaced along the fractures and pathways the growing magma chambers produced during catastrophic expulsion of the saline hydrothermal fluids.
That abundant saline hydrothermal fluids were associated with these intrusives is evident from the hydrothermal alteration present especially in the quartz syenite bodies, and from the contact metasomatic alteration and brecciation of the sedimentary rocks immediately adjacent to the intrusives (Itamar and Baer 1993). Furthermore, polymetallic hydrothermal mineralization occurs as veins and lenses within phreato-magmatic breccia zones at the roofs of the quartz syenite intrusions close to their contacts with the overlying sedimentary rocks. This polymetallic hydrothermal mineralization consists of Ag, Pb, Zn, Cd, Cu, Co, Ni and Fe sulfides, arsenides and sulfo-arsenides plus native Sn in a gangue-dominated by quartz and abundant anhydrite and gypsum, with rare K-feldspar and fluorite. K-Ar dating of this gangue K-feldspar at 125±2 Ma indicates that this hydrothermal veining was the last stage in the magmatic activity (Itamar and Steinitz 1988). Significantly, the calculated oxygen and sulfur isotopic compositions of the hydrothermal fluids, based on analyses of oxygen isotopes in the gangue quartz and sulfur isotopes in the vein sulfides (Itamar and Matthews 1988), indicate that the hydrothermal fluids and the sedimentary connate waters had the same composition, consistent with mixing of the two. Thus there is sufficient evidence of a causal relationship within the timeframe of the Flood between the hydrothermal fluids generated and expelled by all this magmatic activity and the deposition via precipitation of the sulfates within the Ramon Group sediments, particularly the Mohilla Formation.
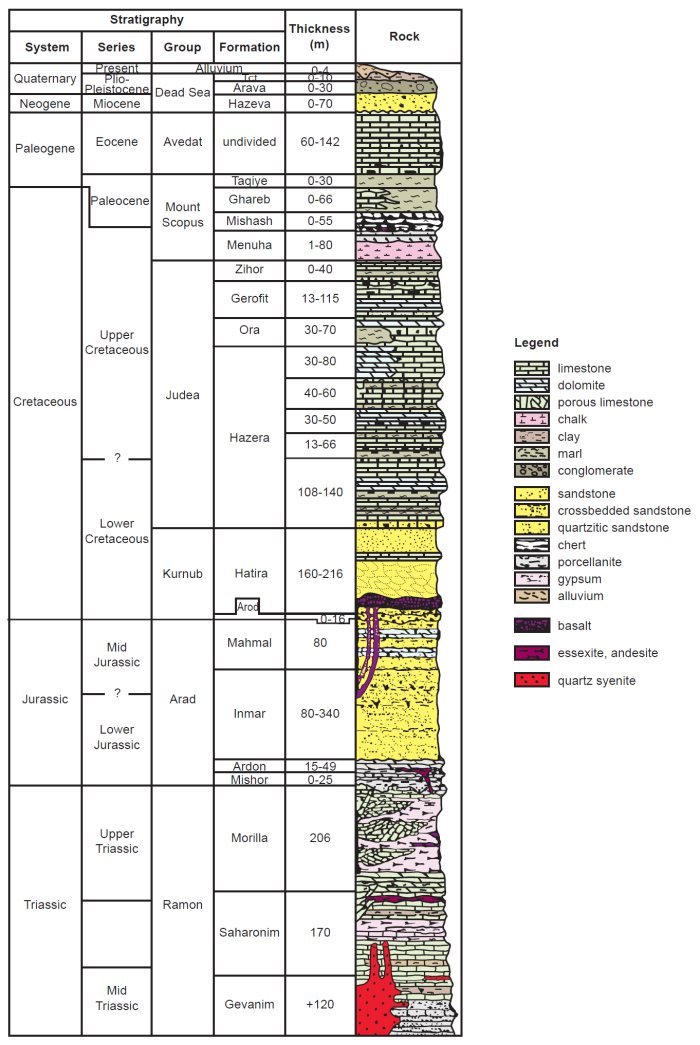
Fig. 15. Columnar stratigraphic section of the layers exposed in the Makhtesh Ramon and Nahal Neqarot areas (after Ben-David 1993).
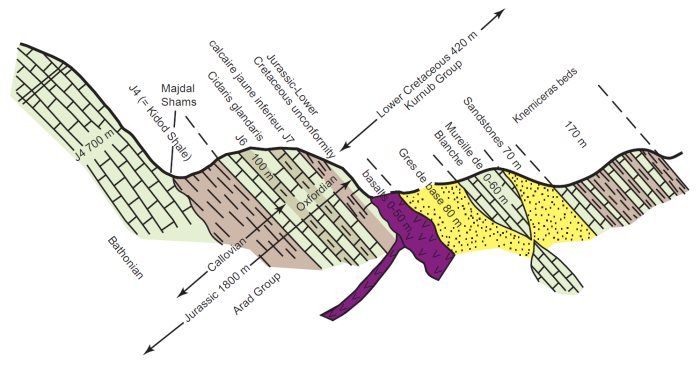
Fig. 16. Generalized stratigraphic section of the upper Jurassic Arad Group and lower Cretaceous Kurnub Group strata sequence exposed in the southeastern slope of Mt. Hermon, northern Israel (after Freund 1978).
Arad Group (Jurassic)
The Jurassic rocks of the Arad Group are also exposed in the erosional cirques in the Negev (fig. 15) and in neighboring northern Sinai and Jordan, as well as being encountered in many boreholes (Garfunkel 1978). The stratigraphy in the Negev was established by Goldberg and Friedman (1974), while the paleontology was studied by Hudson (1958). This Jurassic sequence extends into central and northern Israel, being exposed only in a small area in Samaria (Freund 1978), but is widely exposed on Mt. Hermon (figs. 1, 16 and 17) and in Lebanon.
In all places the top of the Jurassic sequence was eroded, this sequence being completely removed in the central Negev, before deposition of lower Cretaceous rocks. The contact with the upper Triassic rocks in the Negev is unconformable, and marks a brief hiatus in deposition. The upper surface of the Triassic rocks was eroded, apparently weathered and covered by a few to 30 m (98 ft) of kaolinitic clays, often with iron oxides, and having a pisolitic structure. These comprise the Mishor Formation (fig. 15). In spite of the claim that this formation was produced by a prolonged weathering episode, it is admitted that at least some of its material was allochthonous (transported into position) (Garfunkel 1978). This formation occurs in a 50 km (31 mi.) wide belt, which is truncated to the south, where it contains dolomite beds consistent with water-transported deposition.
The Jurassic Arad Group sequence of the Negev is divided into the following formations (fig. 15):
- The Mishor Formation, a few to 30 m (98 ft) thick accumulation of kaolinitic clays with iron oxides and a pisolitic structure, and some dolomite beds.
- The Ardon Formation consists of limestone, shale and dolomite, and in the subsurface also contains some evaporites (precipitites).
- The Inmar Formation is mainly sandstones, some with cross-bedding, but in the subsurface further north it contains some shale and carbonate beds. The formation is rich in plant remains and contains a few thin coal beds.
- The Daya (Mahmal) Formation consists of alternating fossiliferous limestones, sandy limestones, and shales and some sandstones. The carbonate sediments are claimed to have been dolomitized subsequent to deposition then dedolomitized, but such claims expose the inability in conventional thinking to satisfactorily explain the process responsible for forming dolomites. It is more likely that these carbonate sediments were deposited as dolomites due to the chemistry of saline hydrothermal fluids mixing with the Flood waters, with de-dolomitization occurring subsequent to deposition as connate waters leached and removed magnesium.
- The Sherif Formation resembles the Daya (Mahmal) Formation but also contains much disseminated pyrite, and carbonized plant remains, as well as coal beds.
- The Zohar Formation consists predominantly of fossiliferous limestone, marl and shale, with subordinate amounts of silt and sand. Locally it contains marine fossil accumulations in structures claimed to be fossilized reefs, but these can be better explained as depositional features (Snelling 2009b). Some dolomitization and de-dolomitization is also claimed to have taken place, but again the evidence can be interpreted as primary dolomite deposition from saline hydrothermal fluids mixing in the Flood waters, followed by post-depositional leaching and removal of magnesium.
The Sherif and Zohar Formations are not exposed in Makhtesh Ramon because of their non-deposition or erosion in that area and further south (Garfunkel 1978). To the north and northwest the original thickness of Jurassic sediments increases considerably from about 1,000–1,300 m (3,280–4,265 ft) in the northern Negev to about 3,000 m (9,842 ft) under the coastal plain. Most of the thickness difference was produced during deposition of the Ardon and Inmar Formations, although in the northern Negev three additional upper Jurassic formations were deposited on top of the Zohar Formation, the uppermost unit of the Arad Group:
- The Kidod Formation consists predominantly of shales with a few carbonate layers. It is rich in pyrite and plant debris, while marine fossils are abundant especially in the limestone beds and lower shale beds.
- The Beer Sheva and Halutsa Formations consist of alternations of fossiliferous limestones, which are sometimes dolomitic, and shales, with subordinate sandstone in the upper part of the section.
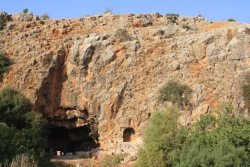
Fig. 17. Upper Jurassic Arad Group limestone at Banias (the biblical Ceasaria Philippi) on the slopes of Mt. Hermon, northern Israel.
Marine fossils are common throughout this Jurassic sequence (Barzel and Friedman 1970; Hudson 1958). These include pelecypods, gastropods, echinoids, crinoids, corals, sponges, brachiopods, ammonites, stromatoporoids, calcareous algae and ubiquitous foraminifers. They are found sporadically scattered throughout the sequence, with some forms more common that others at different levels. Typically they are only preserved as skeletal fragments, such as loose tests, shells, plates, spicules and spines, embedded haphazardly in a micrite or sparite matrix (Barzel and Friedman 1970). Many fossil fragments are coated with algal crusts, and pellets (fecal or mud aggregates) are sporadic. Quartz grains, making up to at least 7% by volume of the fragments embedded in the matrix, are scattered through the rocks. These textural features and this fossil content is fully consistent with rapid water-transported deposition of these rocks.
North of the Negev in central and northern Israel was a domain of continuous calcareous deposition, so there most of these formations (except the upper Jurassic ones) lose their identity (Garfunkel 1978). The Arad Group in northern Israel is composed of limestone with some shale in a 2,000–3,000 m (6,560–9,842 ft) thick sequence (figs. 3 and 16). At the base of the sequence in a downfaulted block in the Carmel area just south of Haifa deep boreholes encountered a volcanic sequence about 2,500 m (8,202 ft) thick consisting predominantly of flows and pyroclastics (Garfunkel 1989). Called the Asher Volcanics, petrographic and geochemical studies have shown that the fresh rocks are alkali olivine basalts (Dvorkin and Kohn 1989), with rare earth elements and Sr and Nd isotopic signatures resembling ocean island and other intraplate basalts, but spilitized rocks are also common. K-Ar dating has yielded ages in the range of about 190–205 Ma (uppermost Triassic–lower Jurassic) for the relatively fresh basalts (Lang and Steinitz 1987), which is consistent with these volcanics overlying upper Triassic limestones.
Kurnub Group (lower Cretaceous)
Cretaceous rocks are exposed very extensively in Israel (figs 1 and 2) and in neighboring regions. They lie unconformably on upper Jurassic to Cambrian rocks, and even on the Precambrian crystalline basement farther south. This unconformity was obviously due to major erosion as a result of the Flood waters temporarily retreating off the region. This coincided with relatively accentuated earth movements (Garfunkel 1978). This makes sense, because by this time in the Flood year such earth movements would be the beginnings of the final phase in which today’s mountains were starting to be built as a result of crustal isostatic adjustments. Earth movements catastrophically raising sections of the earth’s continental crust would cause rapid retreat en masse of the Flood waters as a sheet over wide regions, resulting in massive sheet erosion. Though large volumes of rocks were removed across Israel and beyond, the unconformity at the base of the Cretaceous strata always appears as a smooth surface, both in outcrop and in the subsurface, which is consistent with catastrophic water retreat and sheet erosion (not over 20–30 million years as conventionally claimed).
However, the Flood waters rapidly returned to advance again across the whole of Israel and surrounding regions, progressively depositing a thick blanket of Cretaceous sediments (Sass and Bein 1982) (figs. 2 and 3). In most of the Negev, and especially in outcrops, the lower Cretaceous sequence is predominantly sandstone, which has been designated as the Hatira Formation of the Kurnub Group (Garfunkel 1978) (figs. 3 and 15). Much of this formation consists of variegated, poorly cemented, sometimes cross-bedded, sandstone, which may contain small quartz pebbles, as well as some beds of finely laminated siltstone and marly claystone. The remains of fossil plants are widespread, including fossilized logs exposed by erosion of the Hatira Formation sandstone in Makhtesh Hagadol (fig. 18). In the central Negev the coarse Arod Conglomerate, consisting of quartzite pebbles, occurs at the base of the section (fig. 15). In the nearby eastern Sinai, the Arod Conglomerate is commonly 5 m (16 ft) thick, but ranges from 0–15 m (0–49 ft), as it also does in Makhtesh Ramon (Bartov et al. 1980). The pebbles in it are of various quartzites, reach a size of 30 cm (1 ft) or more, and are embedded in friable sandstone, which is locally limonitic and calcareous at the base.
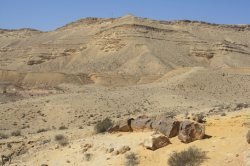
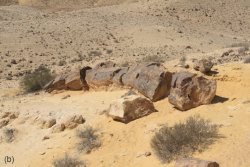
Fig. 18. A fossilized log exposed by erosion from the lower Cretaceous Kurnub Group’s Hatira Formation sandstone on the floor of Makhtesh Hagadol in the Negev, southern Israel. (a) A wide view showing the fossilized log on the floor of Makhtesh Hagadol with the overlying strata exposed behind in the cliffs of the Makhtesh. (b) A closer view of the fossilized log.
These lower Cretaceous Hatira Formation sandstones with the basal Arod Conglomerate are somewhat similar, significantly, to the lower Cambrian Amudei Shelomo Formation of the Yam-Suf Group at the base of the Flood sedimentary sequence, which was deposited by the on-rush of the Flood waters surging onto and over the continents at the beginning of the Flood, similar to, and at the same stratigraphic level as, the Tapeats Sandstone in Grand Canyon (Beus and Morales 2003) and its equivalents across North America (Sloss 1963). However, the Hatira Formation and its basal Arod Conglomerate are the products of what appears to be the last major surge of the Flood waters over the continents prior to the Flood waters finally retreating into today’s new ocean basins. And the presence of one–four interfingering “marine” beds within the Hatira Formation is certainly confirmation of that. The uppermost of these has the greatest extent, reaching the Makhtesh Ramon area 100 km (62 mi.) from the present coast (Garfunkel 1978). These “marine” strata (designated as such because of their contained marine fossils) compromise sandstones, fossiliferous limestones and shales.
Within Makhtesh Ramon angiosperm-like macrofossils and angiospermous pollen grains are found in the lower Hatira Formation sandstones, which also contain marine intercalations with invertebrate fossils, and are topped by the Ramon basalts. The conformable upper Hatira Formation is exposed in the northern slopes of Makhtesh Ramon, and consists of variegated cross-bedded sandstones with lenticular, finely laminated siltstones and marly claystones containing occasional marine fossils and locally abundant terrestrial plant debris. The fossil plant assemblages consist of ferns, ginkgophytes, conifers and the “earliest” angiosperm macrofossils in the stratigraphic sequence (Krassilov et al. 2007). Trunks, roots, fronds and particulate debris of the fern Weichselia are numerically dominant. Next in abundance are narrow angiospermous leaves of several morphotypes, often forming mat-like bedding-plane accumulations that are constantly associated with Weichselia. The other angiosperms are broad-leafed morphotypes, such as the peltate (shield-shaped) Nelumbites or those with sub-peltate platanoid leaves all of which are relatively infrequent, poorly preserved and “apparently” allochthonous (transported), together with occasional leaves and cone scales of araucariaceous conifers. Not only is the evidence that this fossil plant debris was water-transported, but the presence of impressions of insect egg sets on some of the leaf blades (up to 250 eggs on one leaf) indicate transport, deposition, burial and fossilisation had to be rapid, as it would have been under Flood conditions.
In the subsurface of the very northern part of the Negev, the lower Cretaceous Kurnub Group sequence becomes increasingly “marine” (that is, contains marine fossils), and the amount of shales and carbonates increases considerably at the expense of sandstones (Garfunkel 1978). Under the southern coastal plain the sequence is largely marine. The thickness of the Hatira Formation increases from about 200 m (656 ft) in the central Negev to about 400 m (1,312 ft) in the Hatira cirque to the east, while under the southern coastal plain the lower Cretaceous beds are 1,100 m (3,609 ft) thick. In central and northern Israel this mainly clastic Kurnub Group sequence is 800–1,000 m (2,625–3,280 ft) thick. The upper part of the sequence is exposed in several places in central Galilee, while the whole sequence is exposed on the southeastern slopes of Mt. Hermon (fig. 16) and in a small area of the Samaria (fig. 19) (Freund 1978). The sequence begins with alkaline lavas and tuffs, followed by variegated sandstones with fossilized tree remains (figs 16 and 19). A limestone cliff, referred to as “Muraille de Blanche”, marks the middle of the Kurnub Group, which terminates with about 250 m (820 ft) of yellow fossiliferous marls containing some beds of oolitic iron oxides.
As already indicated, during deposition of the lower Cretaceous Kurnub Group sedimentary rocks there was a brief period of magmatism and volcanism in Israel and neighboring areas (Garfunkel 1978).
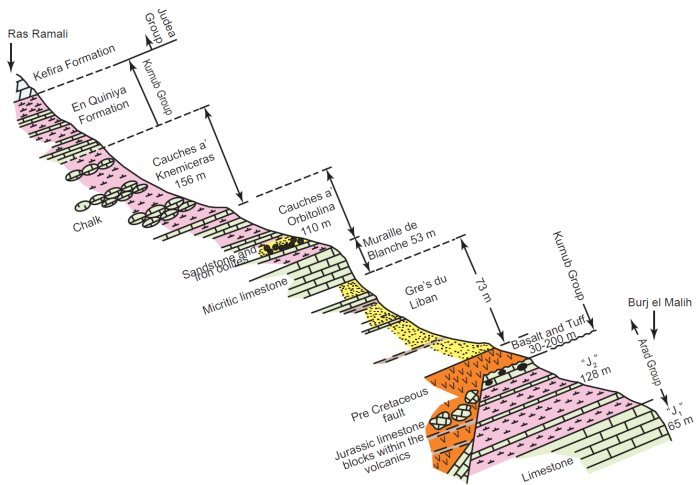
Fig. 19. Columnar stratigraphic section of the upper Jurassic Arad Group and lower Cretaceous Kurnub Group strata exposed at Wadi Malik in Samaria, central Israel (after Freund 1978).
This included the gabbro laccolith, quartz syenite plutons and other intrusions exposed in Makhtesh Ramon in the central Negev, the basalt and trachyte dikes and sills, and the basalt flows referred to above as the Ramon basalt (Baer 1993; Garfunkel 1989; Itamar and Baer 1993; Rophe, Eyal and Eyal 1993). These have been radioisotope dated, yielding various lower Cretaceous ages (Lang et al. 1988; Lang and Steinitz 1985; Lang and Steinitz 1987; Starinsky, Bielski and Steinitz 1980). The intrusions were primarily emplaced in the Triassic Ramon Group and the Jurassic Arad Group, producing metasomatic alteration of the host limestones, for example, in the Saharonim and Ardon Formations (fig. 15). A pavement of the Jurassic Inmar Formation sandstone in Makhtesh Ramon, on a hill known locally as “The Carpentry,” consists of prismatic pillars of hard quartzite, with 3–8 facets, which are 3–12 cm (1.2–4.7 in.) wide and 20–80 cm (7.9–31.5 in.) long (fig. 20) (Mazor 1993). These pillars occur in beds with a total thickness of about 6 m (20 ft), outcropping along 60 m (197 ft). This and other such “carpentries” in the Inmar Formation within the Makhtesh Ramon occur near emplaced magmatic bodies, but they have no direct contact with the pillars, so it has been suggested that these quartzitic pillars were formed by hot fluids that accompanied the igneous intrusions infiltrating into the sandstone. The basalt dikes may have been the conduits from which the Makhtesh Ramon basalts flowed (fig. 15), interrupting deposition of the Hatira Formation sandstones of the lower Cretaceous Kurnub Group. The Arod Conglomerate at the base of the Kurnub Group also contains trachyte pebbles eroded from the trachyte dikes (Garfunkel 1989). The lower Cretaceous basalts seem to have only covered a relatively small area in the central Negev, and neighboring east Sinai (Bartov et al. 1980), but a small basalt plug intruded into Cambrian beds at Timna has a lower Cretaceous K-Ar age (Beyth and Segev 1983), suggesting these basalt flows may have originally extended much further southwards.
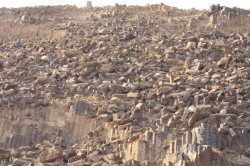
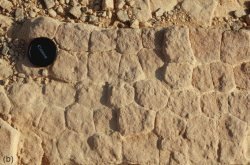
Fig. 20. The “pavement” of upper Jurassic Arad Group Inmar Formation sandstone in Makhtesh Ramon known locally as “The Carpentry.” (a). A wide view showing the vertical prismatic pillars of hard quartzite (baked sandstone), with 3–8 facets, in beds about 6 m (20 ft) thick. (b) An end on view showing that most of the pillars have 5–6 facets, and are generally about 6–8 cm (2.4–3.2 in.) wide.
In the Samaria-Galilee area, considerable magmatism also occurred, known mainly from the subsurface (Garfunkel 1989). Drillholes which reached below the Cretaceous sequence penetrated up to 400 m (1,312 ft) of extrusives, mainly olivine basalts and tuffs, known as the Tayasir Volcanics. They also outcrop in Wadi Malih in the Somron area, some 10 km (6 mi.) west of the Jordan Valley in northeastern Samaria, where they are 230 m (755 ft) thick (fig. 19) (Freund 1978; Lang and Mimran 1985; Mimran 1972). Within the tuffs are thin beds of laminated shales that are slightly calcareous and contain plant remains, well-preserved skeletons or prints of fish up to 10 cm (4 in.) long, fossil tadpoles and ostracodes. The eastward extension of this volcanic field, offset by the Dead Sea transform fault, is exposed in the south of Mt. Hermon (Garfunkel 1989). There numerous small basalt intrusions cross the upper Jurassic beds, extrusives occur at the base of the lower Cretaceous Kurnub Group sequence (fig. 16) (Freund 1978), and several vents delimited by faults are present (Garfunkel 1989). Geochemical studies show these basalts range from thoeliitic to alkaline and form a typical intraplate suite with a geochemical signature similar to ocean island basalts. K-Ar dating of rocks from both the Wadi Malih and Mt. Hermon outcrops yielded uppermost Jurassic to lower Cretaceous ages (Lang and Mimran 1985; Shimron and Lang 1988).
Judea Group (middle Cretaceous)
The middle Cretaceous sedimentary units of the Judea Group are widely exposed in southern Israel, where in Makhtesh Ramon in the central Negev they are collectively up to 520 m (1,706 ft) thick (fig. 21). To the north of the Negev, outcrops of the Judea Group form the backbone of the mountains of Israel, where the group is about 800 m (2,625 ft) thick and dominated by dolomite. There are facies changes laterally, so that the stratigraphic subdivisions and their names have been defined differently in the Negev (fig. 21) compared with in the Judean Hills to the north (fig. 22).
In the Negev, the Judea Group sequence has been divided into the following formations (fig. 21) (Avni 1993; Bartov, et al. 1972; Bartov and Steinitz 1977; Garfunkel 1978):
- The Hazera Formation consists predominantly of fossiliferous limestone, dolomite and marl. It has been subdivided into five members. The transition between the sandstones of the Hatira Formation on which the carbonate sequence of the Hazera Formation always sits is quite abrupt. Compared with the Hazera Formation sequence in the central Negev (in the Makhtesh Ramon area) towards the south, especially in the Elat area, shale and sandstone become increasingly abundant. To the north and northwest the sequence (especially its lower part) becomes thicker and increasingly dolomitic. Thus near the Dead Sea, in Judea and under the southern coastal plain it consists of a predominantly dolomitic sequence, with some sandstone in the latter region (Arkin and Hamaoui 1967).
- The predominantly marly Derorim Formation is only developed in part of the northern Negev, and is characterized by a rich ammonite fauna.
- The Shivta Formation overlies the Derorim Formation, or the Hazera Formation where the latter is absent. It consists of poorly bedded fossiliferous limestones, occasionally with chert concretions. It often contains fossil rudists, which are large horncoral-like pelecypods (bivalve molluscs) (Moore, Lalicker and Fischer 1952), especially in its upper part where other fossils are also common.
- The Nezer Formation consists of well-bedded limestone, mostly micritic, and occasionally contains sandstones.
- The Ora Formation, developed only in the Makhtesh Ramon area and to the south, consists mainly of marl and shale with some limestone interbeds. Oolitic limestone, gypsum and sandstone occur near its top. Its basal beds are rich in and often packed with ammonites, as seen in the “Ammonite Wall” exposed in the southern side of Makhtesh Ramon (fig. 23). This dramatic display of large ammonites all lying flat and regularly spaced at the same level in the same upturned bed is clearly testimony to their catastrophic transport and burial by the Flood waters, as well as to the rapid deposition of the argillaceous dolomite bed that encloses them. These basal beds are equivalent to the Derorim Formation, while higher ammonite-bearing beds and the overlying parts of the Ora Formation which contain them are the lateral equivalents of the Shivta Formation.
- The cliff-forming Gerofit Formation (fig. 24) overlies the Ora Formation, and consists predominantly of limestone, dolomite, and minor chert, marl and shale. Sometimes this formation contains “banks” of accumulated fossil rudists, with fossil hydrozoa, gastropods (fig. 25) and other pelecypod fragments present, that have been interpreted as “bioherms” (Bartov et al. 1972), but instead would be the result of the rapid pile-up of such broken organic debris by the Flood waters.
- The Zihor Formation occurs above the Gerofit Formation only in the southern half of the Negev (Lewy 1975). It consists of a variety of fossiliferous limestones, marls, sandy limestones and some dolomite. The dolomite is coarse-grained and sandy, and like the sandy limestones often exhibits depositional structures such as planar crossbedding and ripple marks (Bartov et al. 1972), which are consistent with clastic deposition by the fast-moving Flood waters. The Zihor Formation forms a soft landscape above the cliffs of the Gerofit Formation. Some confusion has existed over its classification. Because it resembles the underlying beds and its top is an unconformity, it is usually included in the Judea Group. However, due to its claimed fossil age, where its upper boundary is indistinct it has sometimes been included in the overlying upper Cretaceous–Paleocene Mt. Scopus Group.
The fossiliferous sections in the lower Judea Group sequence in the Negev contrast with the dolomite-rich sections north of it, indicating different depositional conditions and source materials. The sandstone occurrences are compatible with sediment transport from the south and southwest (Garfunkel 1978). Sedimentation patterns then changed in response to differential subsidence, so that by the time the upper Judea Group was deposited the northern part of the Negev had become a relatively uplifted area, on which reduced thicknesses of sediments were deposited. South of it much thicker sections accumulated in a relatively subsiding area. There was an influx of clastics, so argillaceous sedimentation extended over much of the Negev. The occurrences of fossil ammonites seem to outline several depositional “belts”, which have been interpreted as a result of structurally controlled depressions in which the waters were deeper than in nearby areas (Freund 1961). However, these belts in the Negev may not have just been associated with marked thickness variations, as facies changes may also have been involved, such as the calcareous sedimentation in the northernmost Negev and beyond, in contrast to the marly-shaly sedimentation in the central and southern Negev. The distribution of upper Judea Group sandstones indicates a southwesterly provenance.
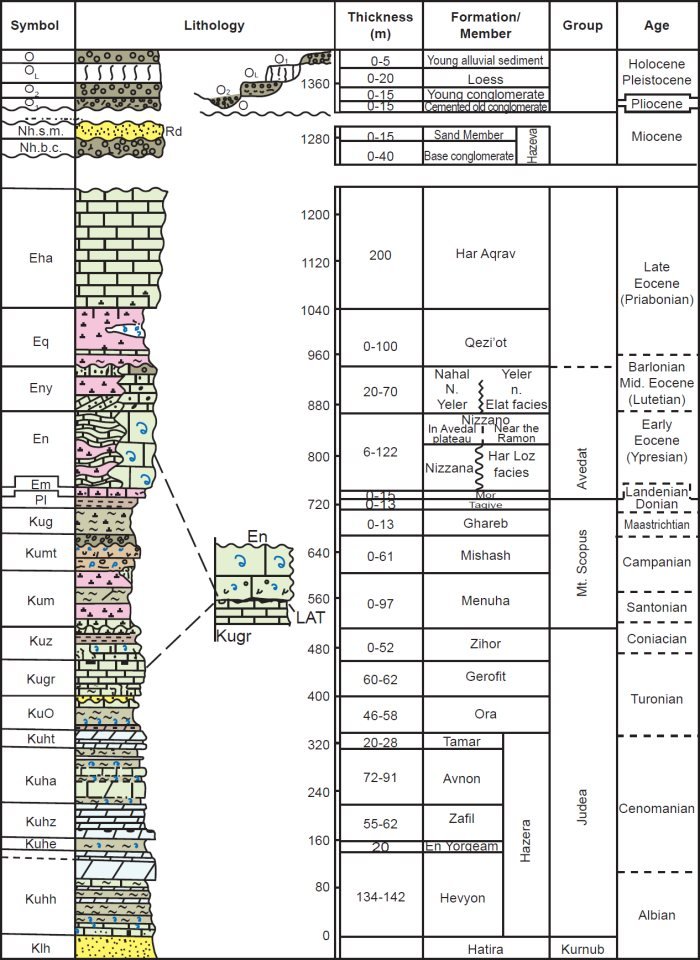
Fig. 21. Composite stratigraphic section for the western area of Makhtesh Ramon with a detailed legend (p. 143) (after Avni 1993). The hard strata of the thick middle Cretaceous Judea Group are prominent.
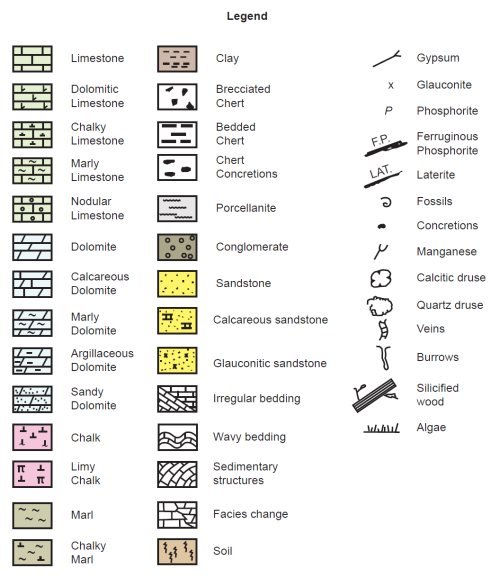
Fig. 21. Legend.
North of the Negev, the Judean Hills, together with the Hebron Hills to the south of them and Samaria further to the north, form the central hilly area of Israel. Outcrops of the Judea Group form the backbone of this hilly area, where the group is about 800 m (2,625 ft) thick and dominated by dolomite. Hard, pure, white, very fine-grained, durable limestone in the Judea Group has been valued for three millennia as a building stone, being used to construct Solomon’s Temple. Much of Jerusalem itself sits on the uppermost beds of the Judea Group, including the Temple Mount (fig. 26). The rock units making up the Judea Group in the Judean Hills are represented schematically in Fig. 22 (Freund 1978; Sass and Bein 1982). The sequence between the Giv’at Ye’arim and Weradim Formations is dominantly dolomitic, but displays distinct vertical and lateral facies changes, no doubt due to the controls on sedimentation, such as water depth and sediment supply.
The variety of dolomitic rocks in the Judean Hills area can be classified into two main facies, which tend to occur in separate formations. First, there are the thickly bedded to massive, coarse to medium crystalline dolomites which occur in the Giv’at Ye’arim, Kesalon, Amminadev and Weradim Formations. Features such as dedolomitization, transitions to limestones and chalks, association with coarsely crystalline silicified rocks, and karstic features are common to these formations. Second, there are well bedded, finely crystalline dolomites which characterize the Soreq and Beit Me’ir (western facies) Formations. These formations are usually poor in calcite, include varying amounts of interbedded clays and marls, and contain siliceous rocks in the form of chert nodules and quartz geodes.
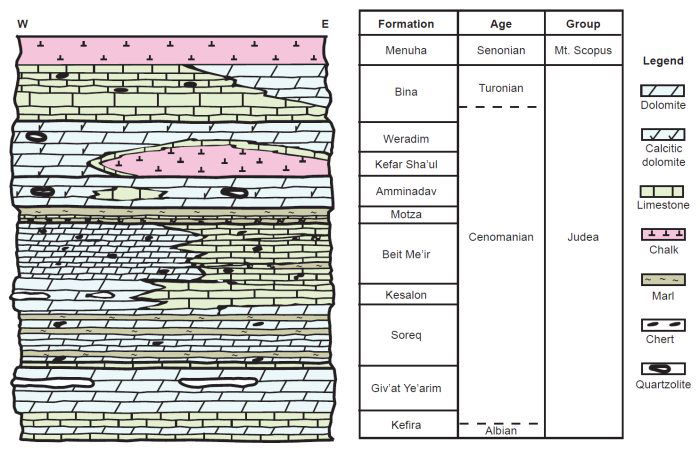
Fig. 22. Lithostratigraphic relationships within the middle Cretaceous Judea Group strata in the Judean Hills (after Sass and Bein 1982). Limestones and dolomites predominate.
Three distinct types of siliceous rocks are closely associated with specific carbonate facies, and thus seem to be related to the depositional conditions. First, there are coarse to medium crystalline silicified rocks termed quartzolites (fig. 22). These usually contain well-preserved skeletal fragments, where the fossil fragments are silicified either selectively or differently from the matrix. On the basis of textural and mineralogical criteria, the formation of these quartzolites and their crystal fabrics is considered to be early diagenetic (Sass and Bein 1982). They are characteristically associated with the coarsely crystalline dolomites. Second, chert occurs as nodules and thin layers, and is quite common in the Soreq and Beit Me’ir Formations (fig. 22). Cherts are rarely associated with the quartzolites, indicating different modes of formation. Third, there are quartz geodes which contain minor anhydrite inclusions, with relics of original anhydrite nodules. They occur sporadically in, and are a characteristic of, the Soreq and Beit Me’ir Formations, and thus are only associated with the finely crystalline dolomites.
The Motza Formation (fig. 22) marks a stratigraphic break between the underlying sequence of dominantly finely crystalline, well-bedded dolomites and the overlying coarsely crystalline dolomites. It is the only non-dolomitic unit in the Judea Group with a widespread areal distribution, consisting mainly of marl and claystone, with some limestone intercalations and rich marine fossil assemblages.
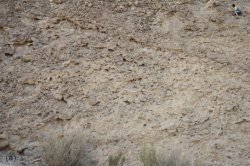
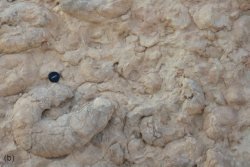
Fig. 23. The “Ammonite Wall” consists of a fossil graveyard of large ammonites on an exposed surface of upturned Ora Formation marl (middle Cretaceous Judea Group) in the southern side of Makhtesh Ramon. (a) A general view of the wall, with a boy for scale in the top right corner. Hundreds of regularly spaced fossilized ammonites can be seen. (b) A closer view of several of the fossilized ammonites. Since the lens cap is 5 cm (2 in.) across, many of these ammonites are 30–48 cm (12–19 in.) across, although there are smaller ones visible. Since these are all the same species in a range of sizes, these represent a living population that perished in a catastrophe, being buried en masse.
Some of the formations display characteristic facies changes, such as the Kefar Sha’ul Formation, which is chalk in the central and eastern Judean Hills, but is calcitic dolomite to the west (fig. 22). Generally speaking, dolomitic facies are better developed in the western Judean Hills, while limey facies are more abundant in the central or eastern part. Because of the observation that dolomites only form today in shallow water evaporitic environments (Kendall 1992) it is claimed that when these dolomites in the Judea Group were deposited the area must have constituted a wide shelf lagoon covered only by shallow hypersaline sea waters (Sass and Bein 1982). Furthermore, relatively deeper waters supposedly existed at different times and places to explain the lateral facies changes from dolomites to chalks and limestones. The diversity of skeletal fossil forms in the chalks and limestones, as well as the planktonic foraminifers and ammonites, is said to indicate close-to-normal salinities prevailed in those depositional areas. However, it is argued here that the dolomites, cherts and anhydrite in the quartz geodes can be better explained as precipitites, whereby contemporaneous magmatic and volcanic activity (for which there is much evidence throughout Israel) contributed copious quantities of hot saline waters and hydrothermal fluids to the cooler Flood waters, that consequently became supersaturated in salts, resulting in deposition of precipitites (Snelling 2009b). Under such Flood conditions the lateral and vertical facies variations in the Judea Group would have resulted from rapid fluctuations in the supply of sediments and salts, and the fluctuations and oscillations in the levels, volumes and flow rates of the Flood waters moving over the continental plates, as they too moved rapidly across the globe due to catastrophic plate tectonics.
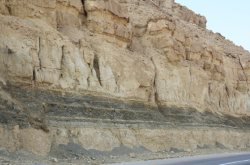
Fig. 24. The cliff-forming Gerofit Formation of the middle Cretaceous Judea Group, as seen here above the highway just below the northern rim of Makhtesh Ramon. The light-colored strata are limestones and dolomites, whereas the dark-colored layers are shale.
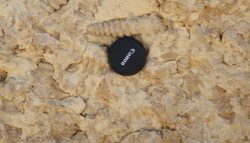
Fig. 25. Fossilized coiled gastropods (marine snails) in a slab of Gerofit Formation limestone (middle Cretaceous Judea Group) on display outside the Makhtesh Ramon Visitors Center. For so many of the one species to be buried together en masse like this in a fossil graveyard is again evidence of catastrophic burial.
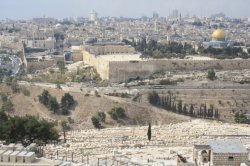
Fig. 26. The Temple Mount (Mt. Moriah), Jerusalem, as seen from the Mount of Olives. The golden Dome of the Rock can be seen top right, and the southeastern corner of the wall of the Old City to the left, with the Kidron Valley below. The Old City is built on the uppermost beds of limestones and dolomites of the Judea Group (middle Cretaceous), which are exposed beneath the wall. The boundary with the overlying Mt. Scopus Group chalk beds is in the Kidron Valley.
Of particular significance is the presence of fossilized dinosaur tracks in the Soreq Formation (fig. 22) at Beit Zeit, a few kilometers west of Jerusalem (Avnimelech 1962, 1966). Over an 80 m2 (860 ft2) area, in the top of an exposed pavement of dolomite, are more than 20 footprint impressions in a continuous row almost 20 m (66 ft) long (fig. 27a). They belong apparently to a single individual. On both sides of this row there are more prints, smaller and less distinct. Each of the footprints in the row show three toes, of which the middle one is 24–26 cm (9–10 in.) long, while the side toes average 20 cm (8 in.) length (fig. 27c). The angle between the toes is about 40°. The distance between the successive alternate footprints is about 80 cm (31 in.) (fig. 27b), so that the distance between one print and the next made by the same foot is around 160 cm (63 in.) or 1.6 m (5.2 ft). Evidently the animal was a bipedal dinosaur, with long and strong hind-feet and probably short fore-feet. On the basis of these data it has been concluded that the hind legs of this theropod dinosaur were approximately 120 cm (47 in.) or 1.2 m (4 ft) high, and that the length of this individual’s entire body with its big tail and expanded neck was 2.5 m (8 ft) or more, making its normal erect posture about 2 m (6.6 ft) tall.
It is because of these fossilized dinosaur footprints that it is envisaged the Soreq Formation dolomites, with minor marls and cherts, were deposited in very shallow water under evaporitic conditions. However, such slow-and-gradual depositional conditions today do not preserve footprint impressions. Nor would dinosaurs have lived in shallow salty water where there was no food to eat! Moving shallow water today will degrade the “walls” of such impressions soon after being made in wet dolomitic sands and muds, and any prolonged period of exposure would obliterate them. On the other hand, the making of these fossilized dinosaur footprints can be explained under the prevailing conditions during the Flood (Snelling 2010b). As already indicated, the dolomitic sands and muds would have been precipitated from hot Mg-carbonate-rich hydrothermal fluids, mixing with the colder Flood waters. During a very brief tidal drop in the water level, this theropod dinosaur (that had earlier been swept away in the Flood waters, in which it was then floundering) was able to walk across a rapidly and temporarily exposed (or semi-exposed) surface of the dolomitic sand/mud leaving its footprints behind. That surface would have been firm due to the cohesiveness of the semi-wet dolomite, where a chemical reaction would start to “set” the dolomite, just as occurs today in very similar man-made cement, retaining the footprint impressions. However, this would have occurred in the brief timeframe before the next tidal surge raised the water level again and swept away the dinosaur, and rapidly covered the footprints with more dolomitic sediments to preserve them. This entire sequence had to have occurred within hours, with its rapid burial and with hardening of the dolomite pavement completed by the weight of the overlying layers squeezing the water out of it, or else these dinosaur footprints would not have been fossilized. Nothing like this happens under today’s conditions. And if this shallow water evaporitic depositional environment had been proximal to where this dinosaur supposedly lived, its bones should be found buried nearby. On the contrary, this dinosaur was swept away in the Flood waters to eventually perish, any trace of its bones likely being buried far away from its footprints, and much higher in the rapidly deposited strata sequence (Brand and Florence 1982; Snelling 2009b).
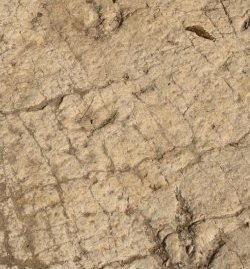
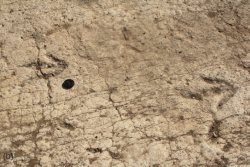
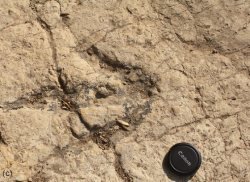
Fig. 27. Fossilized dinosaur footprints in a trackway in an exposed pavement of Soreq Formation dolomite (middle Cretaceous Judea Group) in the village of Beit Zeit, just a few kilometers west of Jerusalem. (a) Three of the 20 or more fossilized footprints in the trackway, a right-left-right set in the direction of walking. (b) A closer view of two of these fossilized footprints, the distance between them being about 88 cm (35 in.). (c) An enlarged view of one fossilized footprint clearly shows the three toes, the middle toe being about 24 cm (9 in.) long and the side toes about 20 cm (8 in.) long. The angle between the side toes is about 40°.
To the northwest of the Judean Hills is an isolated hilly belt of Judea Group strata in the Carmel area south of Haifa (fig. 1). Frequent thickness and facies changes in the strata sequence have made mapping and stratigraphic correlations very difficult. This heterogeneity of facies appears unusual, and is likely due to the area being proximal to the edge of the active deposition of these sediments. The different defined and named rock units in the stratigraphic sequence of the Carmel area is shown schematically in Fig. 28 (Sass and Bein 1982).
Dolomites are again by far the dominant rock units in the Judea Group of the eastern Carmel area, the same types as encountered in the Judean Hills, but with a proliferation of different formation names due to the frequent lateral and vertical facies changes (fig. 28). Those limestones present consist mostly of micrites with fragments of foraminifers, and a few with skeletal fragments of other marine invertebrates. Lenses (50–100 m [164–328 ft] thick and several kilometers wide) of chalk and marl occur in the dolomites, usually with ammonites, echinoids and oysters (Freund 1978). Claimed reef structures and “banks” of fossil rudists, Chondrodonta and Nerinea, which are large horn-coral-like pelecypods (Freund 1978; Moore, Lalicker and Fischer 1952), are here present throughout the entire sequence in various forms (fig. 28). Further to the west the rock units consist mainly of limestones and chalks with some chert. These limestones are mostly calcareous muds (calcilutites) made up of minute allochthonous (transported) skeletal debris, and occasionally foraminifers become an abundant constituent. “Banks” of fossilized oysters are often interbedded in the limestones.
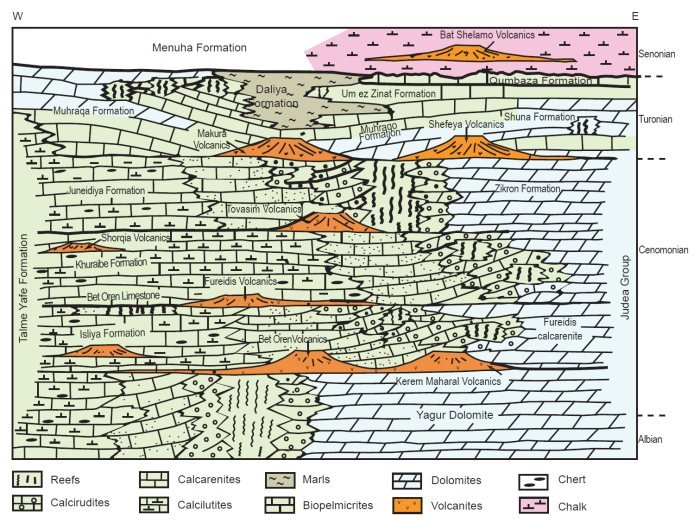
Fig. 28. Lithostratigraphic relationships within the middle Cretaceous Judea Group strata in the Mt. Carmel area south of Haifa (after Sass and Bein 1982). Though dominated by limestones and dolomites, there are frequent intertonguing lateral and vertical facies changes, locally interbedded volcanics, and some claimed “fossil reef” structures that simply represent mounds of limestone debris with fossils (see figs. 29–32).
Intertonguing with the Judea Group even further to the west along the coast is the Talme Yafe Formation (Bein and Weiler 1976; Sass and Bein 1982) (fig. 28). This unit is a huge prism-shaped accumulation (more than 3,000 m (9,842 ft) thick, about 20 km (12 mi.) wide, and at least 150 km (93 mi.) long) of a homogeneous sequence of calcareous detritus deposited primarily as calcilutites (calcareous mudstones) and laminites (turbidites), which are made up of alternating calcilutite and fine calcarenite (calcareous sandstone) laminae. Thin chert horizons are quite abundant. The calcareous detritus consists of minute skeletal fragments of rudistids, echinoids, abraded foraminifers and probably various molluscs, and of carbonate rock clasts. The residue is mostly clays, and siliceous faunal remains such as sponge spicules. Calcirudites (calcareous conglomerates) are found at the base of the sequence. The main extension of these sediments is found in the subsurface of the western part of the coastal plain and offshore, and a small part is exposed in the northwestern Carmel area. This prism (or wedge) is interpreted as being deposited off the continental margin of the northwestern Arabian Craton (Israel) on the continental slope and beyond at its base, the transport of all this carbonate debris from the shelf platform over the edge onto the slope probably being done by storms and tidal currents. Downslope movement would have been in water layers with suspended sediments (debris flows) and gravity-induced (turbidity) currents.
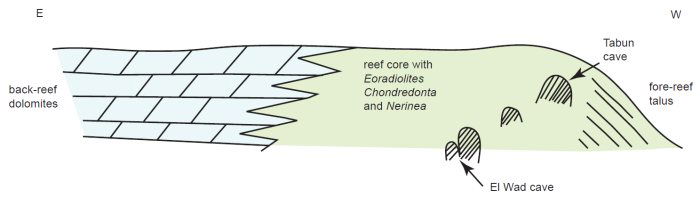
Fig. 29. Generalized north-facing cross-section through the claimed Nahal Hame’arot “fossil reef” complex in the upper Judea Group (middle Cretaceous) strata of the southwestern Carmel area (after Freund 1978). Note that this is only one possible interpretation of the outcrop. Karstic caves are depicted in this north-facing cliff face (see fig. 31), the most famous of which is the Tabun cave where Neanderthal remains were found above stone tools in the sediments on the cave floor.
All the carbonate clastic materials and the tiny skeletal fragments in the thick Talme Yafe Formation are claimed to have been derived from the rudistid “reefs” built on the edge of the continental platform, often as barriers that accumulated dolomites and limestones behind them across the platform. Many other similar examples are found around the world (James and Bourque 1992). But were these really barrier and platform reefs that therefore required countless years to be built, a timeframe inconsistent with the global Flood year? A typical good example of one of these rudistid reef structures is found at Nahal Hame’arot, near the southern end of the Carmel Hills (Freund 1978) (figs 29 and 30). It is said to consist of a rudist Chondrodonta and Nerinea reef core, fore-reef talus, and back-reef “lagoonal” dolomites (Bein 1976). Also present in this example are karstic caves that were inhabited by early post-Babel human settlers (for example, Neanderthals in the Tabun cave) (figs 29 and 31).
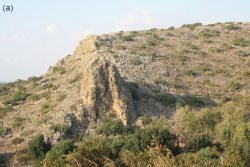
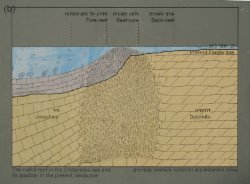
Fig. 30. The south-facing cliff section through the claimed “fossil reef” complex, as exposed by the erosion of the Nahal Hame’arot valley. (a) A view of the actual outcrop. (b) The signboard showing the interpreted “fossil reef” complex. Note that the rugged outcrop with almost vertical sides in the center of (a) is interpreted as the “reef core” in (b), depicted with a jumble of fossilized rudists (the “horn” shapes).
However, the so-called reef core is made up of a jumbled mass of these fossilized rudists (large horncoral-like pelecypods), in places only fragmented rudists, set in a biomicrite matrix, that is, a matrix of fine mud-sized calcareous particles consisting of biological debris derived from the violent destruction of other molluscs, echinoids, ammonites, foraminifers, and more (fig. 32). The “fore-reef” talus consists of biosparites (skeletal fragments set in a lime cement) and biosparrudites (conglomerates made up of biosparite clasts set in a biosparite matrix) which are usually well-sorted and well-rounded and are considered to be reef-debris material that accumulated on the “reef” flanks (Sass and Bein 1982). Such debris beds often dip at about 25°–30°. It is also significant that these so-called reefs only consist of rudists and lack the variety of encrusting organisms inhabiting almost all modern reefs (Bein 1976; James and Bourque 1992). Yet it is claimed that the framework stability of these “reefs” was achieved solely through the “unique growth-pattern” of the rudists (Bein 1976). Such a claim cannot be sustained by observations of the framework construction of modern reefs by numerous varieties of corals, pelecypods, sponges, echinoids and more in growth positions, compared to these rudist-only “reefs” where the rudists are not in growth positions, but are in a jumbled mass cemented by a matrix of biological debris. Thus the evidence emphatically does not support the claim these are grown-in-place reefs. Rather, these are mounds of transported and piled up calcareous debris derived from the violent destruction of other molluscs, echinoids, etc., the larger rudists having survived largely intact by the sorting action of the Flood waters to be buried in these debris piles, all possibly within hours to days due to raging water currents during violent storms.
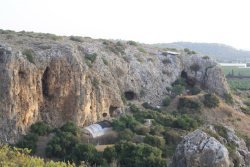
Fig. 31. View of the north-facing cliff section through the claimed “fossil reef” complex (compare with fig. 29). The Tabun cave where the Neanderthal remains were found is the karstic cave on the far right. A man-made roof structure can be seen on the top of the hill above the cave to cover where the cave roof is open.
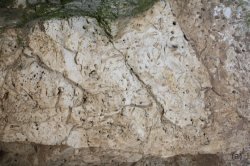
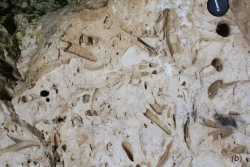
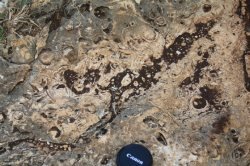
Fig. 32. Fossils in the Nahal Hame’arot “fossil reef” complex. (a) Within the El Wad cave to the far lower left of the Tabun cave (see figs. 29 and 31), the interpreted “reef core” is exposed. Seen here it consists of a jumbled mass burial in a fossil graveyard of large rudists, horn-corallike pelecypods. (b) A closer view of the fossil rudists. The jumbled nature of these horn-shaped rudists is not how they lived. Instead, it is clear they were catastrophically buried en masse by fine mud-sized calcareous particles in a mounded pile. (c) A jumbled mass burial of other molluscs in this same fossil graveyard. This view is of the outcrop just to the right of the rugged section with almost vertical sides in the center of Fig. 30(a), about halfway up the hill, just above the shadow.
There was also contemporaneous volcanic activity in the Carmel area and nearby during all this middle Cretaceous carbonate sedimentation (Sass 1980), which could well have been the source of the hot saline waters that contributed a lot of the carbonates as precipitites. Most of these volcanic rocks consist of mafic pyroclastics, which are associated with basaltic lavas in a few cases only (fig. 1). They form lenticular bodies at various levels in the Judea Group stratigraphic sequence (fig. 28). Three types of pyroclastics rocks have been recognized, each bearing a close relationship to its distance from the eruption center and to its accumulation rate. The first type are black and gray pyroclastics that are usually massive, agglomeratic in places, contain large volcanic bombs and xenoliths, and accumulated in the necks of volcanoes and their immediate vicinities. Next are the variegated pyroclastics, consisting of well-bedded tuffs, lapilli tuffs and agglomerates, containing small volcanic bombs and xenoliths. Their inclination relative to the underlying or overlying beds reaches up to 30°, and their original dips are away from the eruption centers, suggesting these rocks represent the steep flanks of ancient volcanoes, up to 1.0–1.5 km (0.6–0.9 mi.) away from the vents. The maximum thickness of these pyroclastics does not exceed 60 m (197 ft), which has been suggested was controlled by water erosion of the original cones before deposition of the overlying carbonates, meaning the waters at the time were up to 60 m (197 ft) deep across this area. And the third type are yellow tuffs, forming wide, well-bedded blankets which may reach a thickness of 20 m (66 ft), but are usually only a few meters thick. At some locations, marine fossils are in these tuffs, consistent with their distal accumulation.
In the northern part of the mountain backbone of Israel which extends further north into Lebanon, beyond the Judean Hills, is the Galilee region (fig. 1). The area is structurally deformed by gentle folding and intensive faulting which divides the area into a rather complex pattern of horsts, grabens and tilted blocks. The stratigraphic sequence in the Judea Group in the Galilee region is similar to that in the Judean Hills and the Carmel area, but there are also differences due to facies changes. It is schematically shown in Fig. 33 (Freund 1965; Kafri 1972).
The lower part of the sequence, the Kesulat and Yagur Formations, consists of dolomites that are relatively homogeneous in thickness and lithology over the entire area, excluding some claimed local fossil rudist patch reefs. On the other hand, in the upper part of the sequence many facies changes occurred, so the lithologies and thicknesses of the different rock units are both vertically and laterally heterogeneous (fig. 33). The main change is from dolomites to chalky limestones consisting of calcilutites or very fine-grained limestones (the Rosh Haniqra Member of the Sakhnin Formation). Transitional facies, either dolomitic or calcitic (the Ya’ara Member of the Sakhnin Formation, and the Yanuch Formation) are found locally. Simultaneously with the deposition of the upper part of the dolomite section of the Sakhnin Formation, a sequence of claimed rudist reefs (Freund 1965), marls (the Yirka Formation), calcarenites (calcareous sandstones) composed of carbonate rock clasts (the Kishk Formation), and micrites, composed of fine-grained skeletal fragments, was locally deposited.
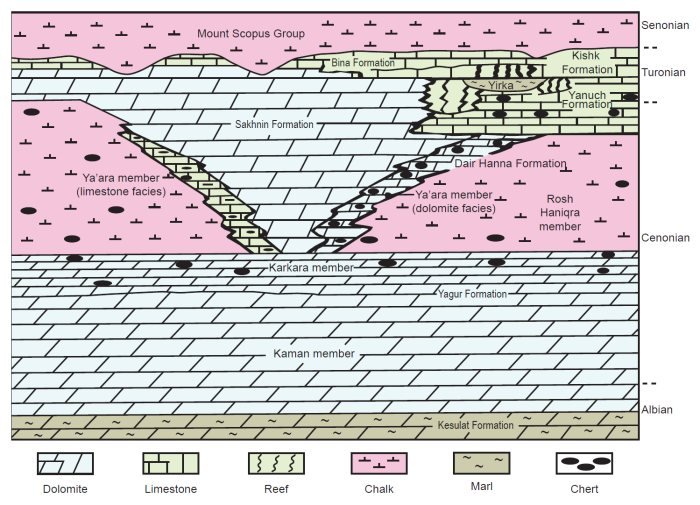
Fig. 33. Lithostratigraphic relationships within the middle Cretaceous Judea Group strata in the Galilee region (after Sass and Bein 1982). Dolomite and chalk beds predominate.
The claimed reef complexes are again open to an alternative Flood interpretation. The long and narrow, massive “reef cores” are surrounded by steep (25°) or gentle (10°) “foreset” beds (Freund 1965). The shells of the “framework builders” (rudists and gastropods) were mostly disintegrated, supposedly due to the boring activity of sponges and algae, so that hardly any of the few rudists (Durania) found are in what might be interpreted as the original growth position. It has even been admitted that these “fossil reefs” cannot be compared with modern coral reefs. The “reef cores” in fact consist of fragmental biogenic limestone, and one of them is capped by a calcareous conglomerate. The claimed “foreset beds” flanking the “reef cores” are in fact cross-bedded pelletal and sandy limestone units that are admitted to have likely formed by erosion and vigorous water currents. Thus the evidence instead favors the interpretation that these so-called reef complexes are in fact simply depositional features due to the rapid and varied actions of the Flood waters, vigorous currents piling up this biogenic and carbonate rock debris.
Mt. Scopus Group (upper Cretaceous–Paleocene)
Overlying the Judea Group locally in erosional and angular unconformity on the east and west sides of the Judean Hills are the “soft” chalk and marl, with some chert beds, of the Mt. Scopus Group. Conventionally these layers are regarded as uppermost Cretaceous to Paleocene (lowermost Tertiary). The Mt. Scopus Group ranges in thickness from 0–500 m (0–1,640 ft) according to the structural position on pre-depositional folds and fault blocks (Freund 1978). It averages about 300 m (984 ft) thick. In Jerusalem the boundary between the uppermost Judea Group limestone beds and the overlying softer chalk beds of the Mt. Scopus Group dips eastward along the Kidron Valley, with the latter beds outcropping on the Mount of Olives to the east of the old city (fig. 34). The chert component in this group increases southwards. There are four formations recognized in the Mt. Scopus Group in the Negev (Garfunkel 1978) (figs. 15 and 21):
- The Menuha Formation, primarily consisting of chalk, disconformably overlies the Zihor or Nezer Formations in the Negev. Thus the stratigraphic position of its base varies (Lewy 1975). Where the formation’s sequence is complete, the middle part contains a bed of phosphate, somewhat sandy, which in the south contains chert and marl. The thickness and stratigraphic scope of this formation strongly depend on its structural position, so that in the Makhtesh Ramon area of the central Negev the formation is from 0–97 m (0–318 ft) thick.
- The Mishash Formation lies conformably on the Menuha Formation, or unconformably on older beds. It is characterized by massive chert beds, accompanied by variable amounts of porcellanite, chalk, marl, claystone, fossiliferous and concretional limestone and phosphorite (Kolodny 1967). Two facies within the formation have been distinguished. The Haroz facies, in which the formation consists of flint only, is developed in part of the northern Negev. It passes laterally into the Ashosh facies in which the additional lithologies are prominent. To the west and northwest the Mishash Formation passes into a continuous chalky facies (Flexer 1968).
- The Sayyarim Formation is the southern equivalent of the Menuha and Mishash Formations (fig. 35). A tongue of chert, marl, limestone and dolomite appears in the Menuha Formation in the southern Negev, and near Elat sandstone (sometimes quartzitic) becomes important. Still farther south the distinct identity of the Mishash Formation is also lost (Bartov and Steinitz 1977).
- The Ghareb Formation consists of yellowish, slightly phosphatic chalk and marl, with minor quantities of dolomite. These rocks are often bituminous. Unlike the underlying formations, this formation’s lithologies are rather uniform over wide areas, though they wedge out over structural highs.
- The lowermost Tertiary (Paleocene) Taqiye Formation is a distinct unit between the Ghareb Formation and the overlying Avedat Group in some locations (Bartov et al. 1972; Bartov and Steinitz 1977; Flexer 1968). The base of the Taqiye Formation, which is up to 50 m (164 ft) thick, is defined as the first appearance of green shales. Calcareous shales and marls, rich in limonite concretions which have a pyritic core, gradually pass upwards into argillaceous chalks and chalky limestones.
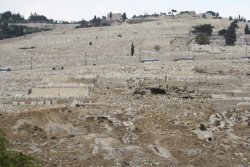
Fig. 34. The Mount of Olives, Jerusalem, looking across the Kidron Valley from beneath the wall of the Old City next to the Temple Mount (fig. 26). The chalk of the Mt. Scopus Group can be seen outcropping in the foreground, just above the boundary with the Judea Group.
The Mt. Scopus Group commonly attains a thickness of 100–200 m (328–656 ft), but variations are common. It predominantly consists of biomicritic, bituminous, poorly-bedded, white foraminiferal chalk, which forms a characteristic landscape of soft hills. Hard calcareous chalks, biorudites and detrital sandy limestones usually occur at the base, and soft white marly chalks and shales terminate the sequence. Flint is abundant, and occurs as massive brecciated brown cliffs or thin continuous or nodular layers. Flexer (1968) distinguished three lithofacies within the Mt. Scopus Group mainly on the basis of the distribution and quantity of flint within the sequence. The Elat lithofacies in southernmost Israel, consisting of chalk alternating with flint, is characterized by large amounts of detrital components, such as quartz sand beds, reworked quartzite and chert pebbles (fig. 35). The Zin lithofacies in the Negev and northwards beyond the Dead Sea area to Galilee is built of chalk and flint beds which gradually intertongue with the pure chalk sequence of the Zefat lithofacies found right along the coastal region of Israel northwards. Certain horizons within the Mt. Scopus Group are very rich in fossil ammonites, lamellibranchs (bivalves), gastropods and sponges, while the chalks are built mainly of foraminiferal tests, ostracods, valves and nannoplankton plates.
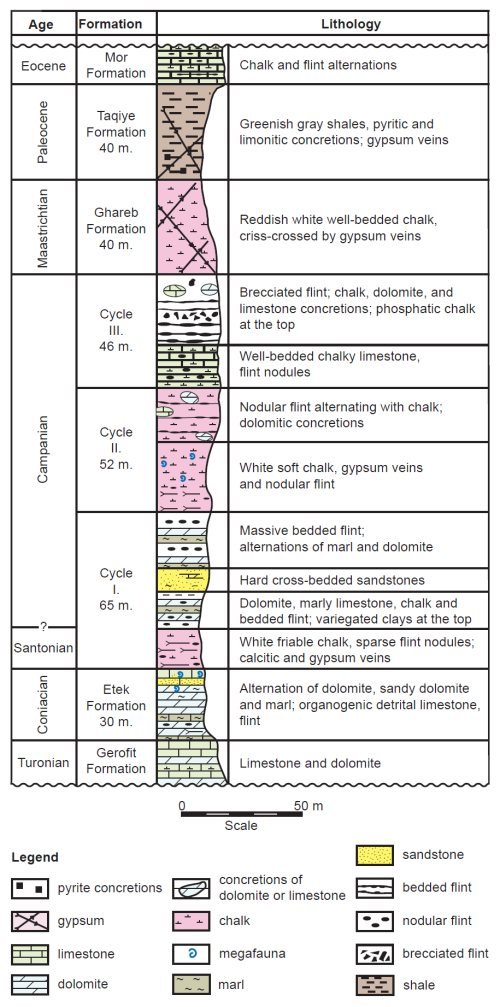
Fig. 35. Composite columnar stratigraphic section of the Mt. Scopus Group in the Elat area in southernmost Israel (after Flexer 1968). The three cycles depicted for the Santonian-Campanian are together named the Sayyarim Formation, the lateral equivalent of the Menuha and Mishash Formations in the Makhtesh Ramon area to the north (figs. 15 and 21).
Of particular interest are the bedded cherts (and flint nodules) within the chalk, and phosphorites of the Menuha, Mishash and Sayyarim Formations in the Negev particularly (Kolodny 1967, 1969; Steinitz 1977). Indeed, cherts, porcellanites and silicified carbonate rocks and phosphorites form the bulk of the upper Cretaceous Mishash Formation. The four main rock types are homogeneous chert, chert spheroids, heterogeneous (brecciated) cherts, and porcellanites (Kolodny 1969). The dominant component of the homogeneous chert is micro- and crypto-crystalline quartz. Silicified fossils are beautifully exposed, ghosts of foraminiferal tests are common, and foraminiferal cavities are infilled with coarser quartz. The chert is usually brown, with the centers of beds or nodules often being black due to the higher (up to 1.3%) content of organic matter. The spheroids vary from almost spherical to disclike, the latter lying parallel to bedding planes, their diameters varying between a few centimeters (<1 in.) and half a meter (19.7 in.). The concentric appearance is caused by alternation of broad (2–5 cm) (< 1–2 in.) brown micro-crystalline quartz bands with thin (0.1–2 mm) (0.0039–0.079 in.) transparent chalcedonic bands. The heterogeneous chert consists of what appear to be chert fragments set in a chert matrix or cement, both components being micro-crystalline quartz. Usually the matrix is finer grained, is much richer in pigmented materials, phosphate detritus and foraminifera, and is more enriched in Ti, Fe, Mg, V and organic matter relative to the fragments. The porcellanite is an impure, usually opaline rock having the texture and appearance of unglazed porcelain. Abundant microfossils in the porcellanite are infilled by micro-crystalline quartz. The porcellanite consists of a-crystobalite (30–80%), the rest being calcite and quartz. Phosphate minerals are abundant throughout the entire Mishash Formation, as are interbedded carbonates (usually sparse biomicrites). The concentration of phosphate increases from the bottom upwards and culminates in the uppermost phosphorite unit. The major phosphate mineral is francolite (apatite, or calcium phosphate, with >1% F and appreciable CO2), which occurs as bone fragments and pellets. The cement is calcite (micrite or sparite), but sometimes is siliceous.
Based on the textures observed in these Mishash cherts, Kolodny (1969) concluded some of the cherts formed by replacement of carbonates (principally chalk), while others precipitated as primary silica, most likely in a silica-saturated environment. Steinitz (1977) reported indications of primary or diagenetic evaporite minerals within the cherts are rare and dispersed, both stratigraphically and geographically. These included the sulfate minerals gypsum and anhydrite (Ca), and celestine (Sr), as well as dolomite (Mg, Ca carbonate). It is thus clear that saline conditions were necessary for both the cherts and these “evaporite” minerals to form. However, it is incorrect to assume these minerals formed by evaporation. Instead, these silica, sulfate and carbonate minerals readily precipitate from saline fluids, particularly hot saline fluids (Hovland et al. 2006). Thus it can be envisaged that these cherts and associated minerals precipitated as saline to saturated hydrothermal fluids, emanating from deep magmas and hot basement rocks via fissures, made contact and mixed with the cooler sediment-carrying Flood waters transgressing the continental crustal surfaces (Snelling 2009b).
These same hot saline to saturated (hydrothermal) fluids are also the key to explaining the rapid Flood accumulation of the chalk beds themselves (Snelling 1994, 2009b). The modern analog for the chalk beds is the calcareous ooze dominated by similar coccoliths now accumulating on the ocean floors at a rate of 2–10 cm (0.79–4 in.) per thousand years (Kukal 1990). At that rate, 200 m (656 ft) thickness of Mt. Scopus Group chalk beds would have taken 2–10 million years to accumulate, which has been cited as an obvious problem for Flood geology (Hayward 1987). However, even today coccolith accumulation is not steady-state but highly episodic, with significant increases occurring in plankton “blooms,” red tides, and in intense white water coccolith blooms in which microorganism numbers experience a two orders of magnitude increase (Seliger et al. 1970; Sumich 1976). Though poorly understood, the suggested reasons for these blooms include turbulence of the sea, wind, decaying fish, nutrients from freshwater inflow and upwelling, and temperature (Ballantyne and Abbott 1957; Pingree, Holligan and Head 1977; Wilson and Collier 1955). There is also experimental evidence that low Mg/Ca ratios and high Ca concentrations in seawater, similar to the levels in so-called Cretaceous seawater from which the chalk beds formed, promote exponential growth rates of coccolithophores (Stanley, Ries and Hardie 2005). Quite clearly, all these necessary conditions for explosive blooming of coccolithophores would have been present during the cataclysmic global upheavals of the Flood. Torrential rain, sea turbulence, decaying fish and other organic matter, and the violent volcanic eruptions on the ocean floor and on land causing steam, carbon dioxide, Ca, and other elements and salts to be spewed into the Flood waters, would have resulted in explosive blooms of coccolithophores on a large and repetitive scale. Furthermore, the ocean water temperatures would have been higher towards the end of the Flood when these Cretaceous chalk beds were deposited because of all the heat released by all the catastrophic, global volcanic and magmatic activity. Thus the rapid production of the necessary quantities of calcareous ooze to form the thick chalk beds in a matter of days to weeks toward the end of the Flood year is realistically conceivable (Snelling 1994, 2009b). Indeed, the extreme purity of the chalk beds, usually >90% CaCO3 (Pettijohn 1957), argues for their rapid deposition and formation, and the chert (and the associated “evaporite” minerals) in them are direct evidence of the hot saline to saturated fluids involved.
However, investigations have shown that once these Mt. Scopus Group chalk beds were deposited the biogenetic fragments were cemented together to make chalky limestone by sparry calcite precipitated from fresh water (Magaritz 1974). This evidence would seem to be contrary to the claim above that the biogenetic debris which constitutes the chalk beds accumulated as a result of the rapid production of coccolithophores in explosive blooms in warm Flood waters being injected with hot saline fluids from violent volcanic eruptions and magmatic activity on a global scale. To the contrary, this fresh water appears to have come from the aquifer below these chalk beds some time after deposition of the biogenetic debris. It is only the lower section of the chalk beds that have been lithified into chalky limestone by the introduction of sparry calcite to infill the foraminiferal tests and pores. And the main indication that lithification was due to sparry calcite precipitated from fresh water is the difference in the oxygen and carbon isotope composition, and the Sr, Fe2O3 and non-carbonate contents, between the chalky limestone and the overlying chalk (Magaritz 1974). But such evidence is not necessarily definitive, and such lithification occurred sometime subsequent to the catastrophic deposition during the Flood, most likely after the Flood waters retreated and the groundwater systems were established by infiltration of post-Flood rainfall.
Avedat Group (Eocene)
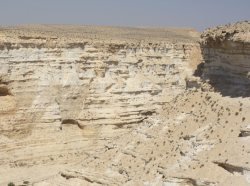
Fig. 36. Cliffs of Avedat Group (Eocene) chalk beds on either side of Wadi Zin at En Avedat, on the northern fringes of the Avedat Plateau in the northern Negev south of Beer Sheva.
Conformably overlying the Taqiye Formation of the Mt. Scopus Group is the Avedat Group, conventionally assigned to the Eocene Series (figs. 15 and 21). Composed of 400–500 m (1,312–1,640 ft) thickness of limestone and chalk beds, the Avedat Group also contains marine fossils. Somewhat harder than the underlying Mt. Scopus Group, it tends to form more resistant ridges and elevated plateaus above the Mt. Scopus strata. Named after the Avedat Plateau south of Beer Sheva (fig. 36) (Bartov et al. 1972), the Avedat Group strata are especially common in structurally low areas, and remnants extend from the Elat area in the south through the Negev to northern Israel. Cliffs of Avedat Group chalk beds occur near Beer Sheva (fig. 37) and also stand beside the valley of Elah (fig. 38) where Goliath challenged the army of Israel, above the brook where David chose five smooth stones (1 Samuel 17).
In the Elat area all four formations within the group, as defined in the Avedat area, can be recognized (fig. 21) (Bartov et al. 1972; Garfunkel 1978). Where the group is complete here it is quite thick at approximately 210 m (689 ft), and consists of chalk, and limestone with variable amounts of chert. Characteristically it is poor in macro-fossils, but is rich in planktonic and benthonic foraminifers. Large foraminifers, like nummulites, are common. The four formations of the Avedat Group in this complete section near Elat are:
- The Mor Formation, 105 m (344 ft) thick, consists mostly of white chalk with black chert occurring in thin to medium lenticular layers. Most of the chert is homogeneous, but some is breccoidal. The chalk often contains dolomite rhombs and phosphate grains, and is silicified. In places, limestone concretions are present within the chalk, their size varying from a few centimeters to 1 m (3.3 ft).
- The Nizzana Formation is 65 m (213 ft) thick, and is composed of alternating yellowish-brown detrital (bioclastic) limestones, phosphoritic limestones, concretionary limestone layers and chalk, with beds and lenses of chert nodules. The limestones are rich in fossil fragments, and sometimes contain macro-fossils. Intraformational conglomerates (calcirudites) and slump structures are common.
- The overlying Horsha Formation, 35 m (115 ft) thick, is composed of white, massive chalk beds with limonitic impregnations topped by variegated shales, alternating with platy chalky limestones. This marly-chalky formation contains some glauconite, today found in marine environments. Its non-carbonate fraction contains clinoptilolite (a zeolite mineral), opal and palygorskite (a clay mineral) associated with montmorillonite (another clay mineral), all indicative of original volcanic components, now altered.
- The overlying Matred Formation is only 15 m (49 ft) thick, and is composed of yellowish, hard, dense and coarse crystalline limestones with abundant nummulites. Chert and some glauconite also occur. The limestones often show cross-bedding, indicative of swift water-current deposition of lime sand in sand waves (Austin 1994).
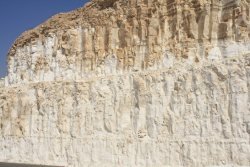
Fig. 37. Thick, massive Avedat Group (Eocene) chalk beds in a road cut just to the northwest of Beer Sheva. Note the purity of the chalk, which is consistent with rapid deposition and accumulation.
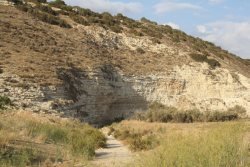
Fig. 38. Laminated Avedat Group (Eocene) chalk beds in the cliffs bordering the Valley of Elah, where Goliath challenged the army of Israel. In the foreground is the brook from where David chose five smooth stones (1 Samuel 17).
The Avedat Group, a 400–500 m (1,312–1,640 ft) thick sequence of lower to middle Eocene “marine” sediments, was deposited in pre-existing synclinal basins (Freund 1978). It lies unconformably, usually with green glauconite beds, on older elevated structures. In the eastern regions of Israel and the Negev the group is dominated by hard limestones composed of benthonic foraminifera, while in the western regions the facies is chalk composed of planktonic foraminifera. This east-west distribution occurs only in the northern part of Israel and not in the Negev, and towards the coastal plain the Avedat Group strata are so chalky they resemble the underlying Mt. Scopus Group chalk beds. Chert is more abundant in the chalky facies in the west than in the limestones to the east and south.
The Flood/post-Flood Boundary
With the widespread deposition of the Avedat Group marine sediments completed, “the continuous marine sequence of the country comes to an end” (Freund 1978). A major regression began in the upper Eocene with the retreating of ocean waters off the country (Garfunkel 1978). Upper Eocene sediments are very rare, mostly being confined to the present coastal plain and bordering foothills. The original extent of these upper Eocene sediments remains unknown, but they could have extended quite a way into structural lows in the Negev (Sakal, Raab and Reiss 1966). For example, there is a small outcrop of upper Eocene Qezi’ot Formation (calcareous muds and clays with marine fossils) overlying the middle Eocene Matred Formation of the Avedat Group in the Menuha anticline area in the southeast Negev (fig. 39). Overlying it on an erosional unconformity is the Miocene Hazeva Formation. Similarly, the Qezi’ot Formation (upper Eocene) also outcrops in the western Makhtesh Ramon area, where it largely consists of chalk (fig. 21). In the same area the Har Agrav Formation (also upper Eocene) marine limestone beds overlie the Qezi’ot Formation. Again, there is then an erosional unconformity above these Eocene strata, with the thin continental sediments of the Miocene Hazeva Formation overlying it.
This regression during and after the upper Eocene was followed by a period of extensive erosion, which produced a rather flat landscape (Garfunkel and Horowitz 1966). Fig. 2 shows the extent of this massive erosional unconformity right across Israel from south to north, apart from some minor continuous deposition of chalk, marl, limestone and shale through the Oligocene on the coastal plain adjacent to the Mediterranean basin, to where the Flood waters would have retreated. Five principal stages in the development of the Negev have been distinguished (Garfunkel and Horowitz 1966), of which the first and the last were mainly erosional, while the others left Miocene continental sediments—the Hazeva Formation which interfingers with Mediterranean marine sediments, the Arava Conglomerate in the deepened Dead Sea rift, and the HaMeshar Formation on wide floodplains. It is noteworthy that these Miocene and later continental sediments lie on rocks which now build the landscape, which shows that many relics of the middle Tertiary topography are still preserved (Garfunkel 1978).
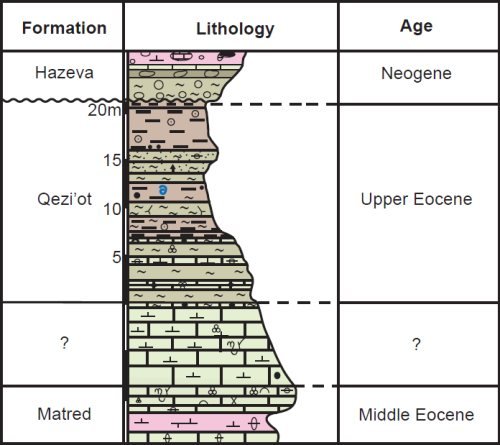
Fig. 39. Generalized stratigraphic column for the Tertiary part of the strata sequence exposed in the Menuha anticline area in the southeast Negev (after Sakal, Raab and Reiss 1966). Note the erosional unconformity above the marine Eocene Qezi’ot Formation, the likely Flood/post-Flood boundary because the Miocene Hazeva Formation above consists of continental deposits.
It is because these Miocene sedimentary rocks above the Eocene “marine” Avedat Group are continental in origin, are of relatively small volume, and are very restricted in extent, that Austin (1998a) implied they are post-Flood. These are the same criteria, namely, continental sediments of relatively small volume and very restricted in extent, that Austin et al. (1994) used to place the Flood/post-Flood boundary globally at the Cretaceous/Tertiary (K/T) boundary in the geologic record. Similarly, Whitmore and Garner (2008) listed criteria such as local sedimentary units, lacustrine and fluvial (continental) deposits, and true desiccation cracks, evaporites and bioturbation as indicative of post-Flood sedimentary rocks. It was using these (and other) criteria that the Eocene Green River Formation of Wyoming was classified as post-Flood (Oard and Whitmore 2006; Whitmore 2006a, b, c; Whitmore and Garner 2008).
However, Whitmore and Garner (2008) also allowed for the residual deposition of marine sediments on the continents after the Flood, presumably in the regressive sequences they list as a criterion for post-Flood sedimentary units. Austin (1998a) also argued for marine sediments still to be deposited on the continents in regressive sequences as the Flood waters retreated, because he stated that:
As the ocean retreated, nutrient-rich waters allowed coccoliths to flourish as massive algal blooms contributed oozes to the ocean floor . . . (so that) marine sedimentation of chalk continued into the post-Flood period in Israel.
The chalk sedimentation he referred to could only be the chalk beds that dominate the Mt. Scopus and Avedat Groups (figs. 2 and 21). However, these groups span the interval from the upper Cretaceous through to the upper Eocene with continuous deposition of thick chalk beds, with some cherts and marls, and minor limestones right across Israel (fig. 2). These certainly represent marine sediments deposited on the continent, but their massive nature (figs. 2 and 3) and fairly uniform thickness right across Israel do not suggest they belong to a regressive sequence.
To the contrary, rather than placing the Flood/post-Flood boundary in the geologic record of Israel at the Cretaceous/Tertiary (K/T) boundary in the middle of where there was continuous chalk deposition, it makes more sense to place it between the Eocene and Miocene. At the end of the Eocene there is a major well-defined and recognized regression right across Israel due to the retreating of the ocean (Flood) waters off the country (Garfunkel 1978), followed by a period of extensive erosion in the Oligocene (Garfunkel and Horowitz 1966), before the arguably post-Flood isolated minor continental sediments were deposited in the Miocene (fig. 2).
Furthermore, the originally continuous Arabo-African craton was only rifted apart in the Cenozoic to form the present plate boundaries in Israel and nearby countries (Garfunkel 1978). Rifting began only in the Oligocene in the southern Red Sea area to begin opening it, but most of the opening of the Red Sea was contemporaneous with the slip on the Dead Sea rift, the majority of which was probably during the Miocene (Freund, Zak and Garfunkel 1968). At the same time this rifting formed the Dead Sea basin, the Jordan valley, the Sea of Galilee and the Hula basin (another lake-filled depression north of Galilee), drag and frictional forces along the Dead Sea Transform Fault caused the thick sequence of Flood strata in central and northern Israel, including the limestone and chalk beds of the middle Cretaceous to Eocene Judea, Mt. Scopus and Avedat Groups, to be arched upward to form the Judean Mountains and the adjoining foothills to the west (Austin 1998a).
According to the biblical account, from Noah’s perspective the Flood event had ended when God told Noah that he, his family and all the animals could leave the Ark (Genesis 8:15–19), 371 days after they had boarded the Ark and the Flood had begun (Genesis 7:11 cf. 8:14). However, the Ark had come to rest on “the mountains of Ararat” 150 days into the Flood event (Genesis 8:3–5), so the Flood waters had been retreating and decreasing off the continental land surfaces for at least 221 days since that time point. Indeed, from Noah’s subsequent visual observations the waters had dried up from off the earth, at least from around where the Ark had landed (Genesis 8:11–13), well before God’s command to disembark from the Ark. Yet even then we cannot say for certain that the Flood waters had fully retreated off today’s land surfaces into the present ocean basins.
Since the geologic processes which were occurring at catastrophic rates during the Flood are still operating today at a snail’s pace (for example, plate tectonics and volcanism), it is likely that as the Flood ended these geologic processes did not stop abruptly, but rapidly decelerated. This is confirmed by the declining eruption power of post-Flood volcanoes (Austin 1998b). Thus it is likely there were residual local catastrophes in the early post-Flood years that produced some sedimentary layers in local basins and dramatically eroded some impressive landscape features. And in some places marine sediments could still have been deposited on continental land surfaces marginal to today’s ocean basins, because the Flood waters had not then fully retreated to today’s coastlines.
It could thus be argued from the above considerations that the Flood/post-Flood boundary in the geologic record of Israel, coinciding with Noah disembarking the Ark, could still be at the Cretaceous/Tertiary (K/T) boundary within the Mt. Scopus Group strata, in keeping with the Austin et al. (1994) positioning of the Flood/post-Flood boundary from a global perspective, because the Flood waters had not fully retreated from off the land of Israel. However, given that there is strong, well-recognized evidence of the ocean (Flood) waters having finally retreated from off the land of Israel to approximately the present coastline immediately after continuous (uninterrupted) deposition of the thick “marine” limestone, dolomite and chalk beds of the middle Cretaceous-Eocene Judea, Mt. Scopus and Avedat Groups, accompanied by subsequent extensive erosion and drying of the land surface, it seems more reasonable to place the Flood/post-Flood boundary in the geologic record of Israel in the Oligocene, or at the end of it depending on which time point coincides with Noah disembarking from the Ark. This placement of the boundary still requires some residual local geologic activity to have rapidly occurred in Israel (tectonic adjustments, erosion, sedimentation and volcanic eruptions) during the early decades of the post-Flood period before post-Babel people migrated into the land ahead of Abraham’s subsequent arrival.
Conclusion
The sedimentary strata that comprise and cover most of Israel provide an obvious record of the Genesis Flood, in keeping with the geologic evidences as outlined by Austin (1994), Snelling (2007), and elaborated on subsequently (for example, Snelling 2008a, b, c). And the geologic record of the Flood in Israel has many similarities to that in the Grand Canyon-Grand Staircase area of the U.S. Southwest (Austin 1994, 1998a).
The major erosion surface at the base of the sedimentary strata sequence which was cut across the Precambrian (pre-Flood) crystalline basement rocks (metamorphics and granites), and which could be called the “Great Unconformity” of Israel, appears to mark the catastrophic passage of the Flood waters as they rose onto the pre-Flood continental surface at the initiation of the Flood event. The ocean (Flood) waters thus rose over the continental land, as evidenced by the myriads of marine organisms buried and fossilized in sediment layers deposited across Israel (Snelling 2008a). Many thousands of meters of “marine” sediments were deposited on a vast scale. Israel appears to have been on the northern margin of part of the pre-Flood continent, with an ocean basin to the north which was a gigantic dumping ground for the northward-thickening wedge of sedimentary strata across Israel (fig. 3) (Austin 1998a). Even Mt. Hermon, the highest elevation in Israel, is composed of limestone beds, containing marine fossils.
The accumulation of this thick sediment sequence was rapid, as evidenced by mass graveyards of fossils (Snelling 2008b), such as the ammonites now exposed in the upturned layer in Makhtesh Ramon (the “Ammonite Wall”). Molluscs (rudists) were not fossilized in a gigantic, organically-bound reef complex near Mt. Carmel, but are distributed within a matrix of fine-grained lime sediment that was transported, “dumped” in a big “heap”, and rapidly buried (Austin 1998a). Lamination and bedding are distinctive of layering of sedimentary rocks without significant evidence of burrowing and disruption features, implying rapid sedimentation, not enormously long periods of slow accumulation.
At the initiation of the Flood when the ocean waters catastrophically rose and advanced over the pre-Flood supercontinent as it broke apart, eroding the crystalline basement, the first sediment layer to be deposited in Israel and widely across surrounding regions was a sandstone with a conglomeratic base, identical to the Tapeats Sandstone in the Grand Canyon whose equivalents were deposited right across North America. Similarly, late in this Flood inundation of Israel the waters were nutrient rich, likely due to the addition of chemical-rich hot waters from associated volcanism, allowing coccoliths to flourish as massive algal blooms that then rapidly accumulated as oozes to become thick chalk beds. These were not just a local phenomenon, as these chalk beds in Israel can be traced west across Europe to England and Ireland, and east to Kazakhstan, with other remnants in the Midwest of the USA and in southern Western Australia. Both these examples powerfully illustrate the global Flood deposition of transcontinental rock layers (Snelling 2008c).
Finally, on Day 150 of the Flood the Ark landed on “the mountains of Ararat”, and 74 days later the Flood waters had decreased sufficiently for “the tops of the mountains” to be seen (Genesis 8:4–5). The formation of such mountains would have required powerful tectonic upheaval processes that overturned and upthrusted sedimentary strata. Simultaneous isostatic adjustments would also have resulted in restoring the continental land surfaces as the Flood waters drained off into new deep ocean basins. In Israel this great regression, as the Flood waters receded and widespread marine sedimentation ended, also coincided with the commencement of the rifting that opened up the Red Sea and the Dead Sea-Jordan River rift valley along the Dead Sea Transform Fault, as well as the uplifting of the Judean Mountains along a north-south axis of folding (the Judean Arch), and the thrust faulting that created Israel’s highest peak, Mt. Hermon (2,814 m) (9,232 ft), all of which marked the end of the Flood event.
References
Arkin, H., and M. Hamaoui. 1967. The Judea Group (Upper Cretaceous) in central and southern Israel. Geological Survey of Israel Bulletin 42.
Austin, S. A. ed. 1994. Grand Canyon: Monument to catastrophe. Santee, California: Institute for Creation Research.
Austin, S. A. 1998a. Geology of the Holy Land. In Israel: Land, nature, and history, Field Study Tour Guidebook, chapter 2. Santee, California: Institute for Creation Research.
Austin, S. A. 1998b. The declining power of post-Flood volcanoes. Acts & Facts 27.8, Impact #302.
Austin, S. A., J. R. Baumgardner, D. R. Humphreys, A. A. Snelling, L. Vardiman, and K. P. Wise. 1994. Catastrophic plate tectonics: A global Flood model of earth history. In Proceedings of the Third International Conference on Creationism, ed. R. E. Walsh, pp. 609–621. Pittsburgh, Pennsylvania: Creation Science Fellowship.
Austin, S. A., and K. P. Wise. 1994. The pre-Flood/Flood boundary: As defined in Grand Canyon, Arizona and eastern Mojave Desert, California. In Proceedings of the Third International Conference on Creationism, ed. R. E. Walsh, pp. 37–47. Pittsburgh, Pennsylvania: Creation Science Fellowship.
Avigad, D., K. Kolodner, M. O. McWilliams, H. Persing, and T. Wiessbrod. 2003. Origin of northern Gondwana Cambrian sandstone revealed by detrital zircon SHRIMP dating. Geology 31:227–230.
Avni, Y. 1993. The structural and landscape evolution of the western Ramon structure. Israel Journal of Earth Sciences 42:177–188.
Avnimelech, M. 1962. Dinosaur tracks in the lower Cenomanian of Jerusalem. Nature 196:264.
Avnimelech, M. 1966. Dinosaur track in the Judean Hills. Proceedings of the Israel Academy of Science and Humanities, Section of Sciences 1:1–19.
Baer, G. 1993. Flow directions in sills and dikes of cauldrons in eastern Makhtesh Ramon. Israel Journal of Earth Sciences 42:133–148.
Ballantyne, B., and B. C. Abbott. 1957. Toxic marine flagellates: Their occurrence and physiological effects on animals. Journal of General Microbiology 16:274–281.
Bartov, Y., Y. Eyal, Z. Garfunkel, and G. Steinitz. 1972. Late Cretaceous and Tertiary stratigraphy and paleogeography of southern Israel. Israel Journal of Earth Sciences 21:69–97.
Bartov, Y., and Y. Arkin. 1980. Geological photomap, 1:500,000, 2nd ed. Jerusalem: Geological Survey of Israel.
Bartov, Y., and G. Steinitz. 1977. The Judea and Mount Scopus Groups in the Negev and Sinai with trend surface analysis of the thickness data. Israel Journal of Earth Sciences 26: 119–148.
Bartov, Y., Z. Lewy, G. Steinitz, and I. Zak. 1980. Mesozoic and Tertiary stratigraphy, paleogeography and structural history of the Gebel Arief en Naqa area, eastern Sinai. Israel Journal of Earth Sciences 29:114–139.
Barzel, A., and G. M. Friedman. 1970. The Zohar Formation (Jurassic) in southern Israel: A model of shallow-marine carbonate sedimentation. Israel Journal of Earth Sciences 19:183–207.
Baumgardner, J. R. 2003. Catastrophic plate tectonics: The physics behind the Genesis Flood. In Proceedings of the Fifth International Conference on Creationism, ed. R. L. Ivey, Jr., pp. 113–126. Pittsburgh, Pennsylvania: Creation Science Fellowship.
Bein, A. 1976. Rudistid fringing reefs of Cretaceous shallow carbonate platform of Israel. American Association of Petroleum Geologists Bulletin 60:258–272.
Bein, A., and Y. Weiler. 1976. The Cretaceous Talme Yafe Formation: a contour current shaped sedimentary prism of calcareous detritus at the continental margin of the Arabian Craton. Sedimentology 23:511–532.
Ben-David, R. 1993. Stages in the evolution of the landscape in Makhtesh Ramon and Nahal Negarot areas. Israel Journal of Earth Sciences 42:189–195.
Benjamini, C., Y. Druckman, and I. Zak. 1993. Depositional cycles in the Ramon Group (Triassic), Makhtesh Ramon. Israel Journal of Earth Sciences 41:115–124.
Bentor, Y. G. 1961. Petrographical outline of the Precambrian in Israel. Bulletin of the Research Council of Israel 10G: 19–63.
Beus, S. S., and M. Morales (eds). 2003. Grand Canyon geology, 2nd ed. New York: Oxford University Press.
Beyth, M., and A. Segev. 1983. Lower Cretaceous basaltic plug in Timna Valley. Israel Journal of Earth Sciences 32: 165–166.
Beyth, M., R. J. Stern, R. Altherr, and A. Kröner. 1994. The late Precambrian Timna igneous complex, southern Israel: Evidence for comagmatic-type sanukitoid monzodiorite and alkali granite magma. Lithos 31:103–124.
Brand, L. R., and J. Florence. 1982. Stratigraphic distribution of vertebrate fossil footprints compared with body fossils. Origins 9:67–74.
Burke, K., and J. U. Kraus. 2000. Deposition of immense Cambro-Ordovician sandstone bodies, now exposed mainly in N. Africa and Arabia, during the aftermath of the final assembly of Gondwana. Geology Society of America Abstracts with Program 32:249.
Choubert, G., and A. Faure-Mauret. 1975. Geological World Atlas, scale 1:10,000,000, sheets 6, 7 (Africa). Parts: UNESCO.
Clark, M. E., and H. D. Voss, 1990. Resonance and sedimentary layering in the context of a global Flood. In Proceedings of the Second International Conference on Creationism, vol. 3, eds. R. E. Walsh and C. L. Brooks, pp. 53–63. Pittsburgh, Pennsylvania: Creation Science Fellowship.
Cooper, G. A. 1976. Lower Cambrian brachiopods from the rift valley (Israel and Jordan). Journal of Paleontology 50: 269–289.
De Witt, M., M. Jeffrey, H. Bergh, and L. Nicolaysen. 1988. Geological Map Sectors of Gondwana, scale 1:10,000,000. Tulsa, Oklahoma: American Association of Petroleum Geologists, and Johannesberg, South Africa: University of Witwatersrand.
Druckman, Y. 1974. The stratigraphy of the Triassic sequence in southern Israel. Geological Survey of Israel Bulletin 64.
Dvorkin A., and B. P. Kohn. 1989. The Asher Volcanics, northern Israel: Petrography, mineralogy, and alteration. Israel Journal of Earth Sciences 38:105–123.
Flexer, A. 1968. Stratigraphy and facies development of the Mount Scopus Group (Senonian-Paleocene) in Israel and adjacent countries. Israel Journal of Earth Sciences 17: 85–114.
Freund, R. 1961. Distribution of lower Turonian ammonites in Israel and the neighboring countries. Bulletin of the Research Council of Israel 10G:79–100.
Freund, R. 1965. Upper Cretaceous reefs in northern Israel. Israel Journal of Earth Sciences 14:108–121.
Freund, R. 1977. Updating the stratigraphic sequence of Israel. Israel Journal of Earth Sciences 26:30–33.
Freund, R. 1978. Judean hills and Galilee regional synthesis of sedimentary basins. In Sedimentology in Israel, Cyprus, and Turkey, Tenth International Congress on Sedimentology, Pre-congress Excursion A1, pp. 1–31. Jerusalem: International Association of Sedimentologists.
Freund, R., I. Zak, and Z. Garfunkel. 1968. Age and rate of sinistral movement along the Dead Sea rift. Nature 220:253–255.
Garfunkel, Z. 1978. The Negev—Regional synthesis of sedimentary basins. In Sedimentology in Israel, Cyprus, and Turkey, Tenth International Congress on Sedimentology, Pre-congress Excursion A1, pp. 33–110. Jerusalem: International Association of Sedimentologists.
Garfunkel, Z. 1980. Contribution to the geology of the Precambrian of the Elat area. Israel Journal of Earth Sciences 29:25–40.
Garfunkel, Z. 1989. Tectonic setting of Phanerozoic magmatism in Israel. Israel Journal of Earth Sciences 38:51–74.
Garfunkel, Z. 2002. Early Paleozoic sediments of NE Africa and Arabia: Products of continental-scale erosion, sediment transport, and deposition. Israel Journal of Earth Sciences 51:135–156.
Garfunkel, Z., and Horowitz, 1966. The Upper Tertiary and Quaternary morphology of the Negev, Israel. Israel Journal of Earth Sciences 15:101–117.
Goldberg, M., and G. M. Friedman. 1974. Paleoenvironments and paleogeographic evolution of the Jurassic system in southern Israel. Geological Survey of Israel Bulletin 61.
Halpern, M., and N. Tristan. 1981. Geochronology of the Arabian-Nubian Shield in southern Israel and eastern Sinai. Journal of Geology 89:639–648.
Hayward, A. 1987. Creation and evolution: The facts and the fallacies, pp. 91–93. London: Triangle (SPCK).
Holmes, A. 1965. Principles of physical geology, rev. ed. London: Thomas Nelson and Sons.
Hovland, M., H. G. Rueslatten, H. K. Johnson, B. Kvanne, and T. Kuznetsova. 2006. Salt formation associated with subsurface boiling and supercritical water. Marine and Petroleum Geology 23:855–869.
Hudson, R. G. S. 1958. The upper Jurassic faunas of southern Israel. Geological Magazine 95:415–425.
Ilani, S., A. Flexer, and J. Kronfeld. 1987. Copper mineralization in the sedimentary cover associated with tectonic elements and volcanism in Israel. Mineralium Deposita 22:269–277.
Itamar, A., and G. Baer. 1993. Polymetallic mineralization related to magmatic and contact metamorphic features, southern Makhtesh Ramon, Israel. Israel Journal of Earth Sciences 42:149–163.
Itamar, A., and A. Matthews. 1988. Carbon, oxygen, and sulfur isotope studies of the hydrothermal mineralization in the Gavnunim Valley, Makhtesh Ramon, Israel. Israel Journal of Earth Sciences 37:91–103.
Itamar, A., and G. Steinitz. 1988. Potassium-argon ages of polymetallic mineralization in the Gavnunim Valley, Makhtesh Ramon. Israel Journal of Earth Sciences 37: 83–89.
James, N. P., and P.-A. Bourque. 1992. Reefs and mounds. In Facies models: Response to sea level changes, eds. R. G. Walker and N. P. James, pp. 323–347. Geological Association of Canada.
Jarrar, G., H. Wachendorf, and H. Zellmer. 1991. The Saramuj Conglomerate: evolution of a Pan-African molasse sequence from south-west Jordan. Neues Jahrbuch für Geologie und Paläontologie, Monatshefte 6:335–356.
Jarrar, G., H. Wachendorf, and D. Zachmann. 1993. A Pan-African alkaline pluton intruding the Saramuj conglomerate, south-west Jordan. International Journal of Earth Sciences 82:121–135.
Kafri, U. 1972. Lithostratigraphy and environment of deposition, Judea Group, western and central Galilee, Israel. Geological Survey of Israel Bulletin 54.
Karcz, I., and M. Braun. 1964. Sedimentary structures and paleocurrents in the Triassic sandstones of Makhtesh Ramon. Geological Survey of Israel Bulletin 39.
Karcz, I., and C. A. Key, 1966. Note on the pre-Paleozoic morphology of the basement in the Timna area (southern Israel). Israel Journal of Earth Sciences 15:47–56.
Karcz, I., and I. Zak, 1965. Triassic paleocurrent pattern in the Har Arif area, southern Israel. Israel Journal of Earth Sciences 14:45–50.
Karcz, I., and I. Zak, 1968. Paleocurrents in Triassic sandstones of ‘Arayif En-Naqa, Sinai. Israel Journal of Earth Sciences 17:9–15.
Krassilov, V., N. Silantieva, M. Hellmund, and W. Hellmund. 2007. Insect egg sets on angiosperm leaves from the lower Cretaceous of Negev, Israel. Cretaceous Research 28: 803–811.
Kendall, A. C. 1992. Evaporites. In Facies models: Response to sea level changes, eds. R. G. Walker and N. P. James, pp. 375–409. Geological Association of Canada.
Kolodner, K., D. Avigad, M. McWilliams, J. L. Woden, T. Wiessbrod, and S. Feinstein. 2006. Provenance of north Gondwana Cambrian-Ordovician sandstone: U-Pb SHRIMP dating of detrital zircons from Israel and Jordan. Geological Magazine 143:367–391.
Kolodny, Y. 1967. Lithostratigraphy of the Mishash Formation (Negev, Israel), Israel Journal of Earth Sciences 16:57–73. Kolodny, Y. 1969. Petrology of siliceous rocks in the Mishash Formation (Negev, Israel). Journal of Sedimentary Petrology 39:166–175.
Kröner, A., M. Eyal, and Y. Eyal. 1990. Early Pan-African evolution of the basement around Elat, Israel, and the Sinai Peninsula revealed by single-zircon evaporation dating, and implications for crustal accretion rates. Geology 18:545–548.
Kukal, Z. 1990. The rate of geological processes. Earth Science Reviews 28:109–117.
Landing, E., S. A. Bowring, K. L. Davidek, S. R. Westrop, G. Geyer, and W. Heldmaier. 1998. Duration of the early Cambrian U-Pb ages of volcanic ashes from Avalon and Gondwana. Canadian Journal of Earth Sciences 35: 329–338.
Lang, B., E. H. Hebeda, H. N. A. Priem, and G. Steinitz. 1988. K-Ar and Rb-Sr ages of early Cretaceous magmatic rocks from Makhtesh Ramon, southern Israel. Israel Journal of Earth Sciences 37:65–72.
Lang, B., and Y. Mimram. 1985. An early Cretaceous volcanic sequence in central Israel and its significance to the absolute date of the base of the Cretaceous. Journal of Geology 93:179–184.
Lang, B., and G. Steinitz. 1985. New K-Ar ages of Mesozoic magmatic rocks in Makhtesh Ramon. Israel Geological Society Annual Meeting, Yotvata, p. 56 (abstract).
Lang, B., and G. Steinitz. 1987. K-Ar dating of subsurface Mesozoic and Cenozoic magmatic rocks in Israel. Geological Survey of Israel Report GSI/8/87.
Lewy, Z. 1975. The geological history of southern Israel and Sinai during the Coniacian. Israel Journal of Earth Sciences 24:19–43.
Magaritz, M. 1974. Lithification of chalky limestone: A case study in Senonian rocks from Israel. Journal of Sedimentary Petrology 44:947–954.
Mazor, E. 1993. Introduction to the Ramon Geological Park. Israel Journal of Earth Sciences 42:103–114.
Mimram, Y. 1972. The Tayasir volcanics. Geological Survey of Israel Bulletin 52.
Moore, R. C., C. G. Lalicker, and A. G. Fischer. 1952. Invertebrate fossils. New York: McGraw-Hill Book Company.
Oard, M. J., and J. H. Whitmore. 2006. The Green River Formation of the west-central United States: Flood or post-Flood? Journal of Creation 20, no. 1:46-49.
Parnes, A. 1965. Note on Middle Triassic ammonites from Makhtesh Ramon (southern Israel). Israel Journal of Earth Sciences 14:9–17.
Parnes, A. 1971. Late Cambrian trilobites from the Timna area and Har Amram (southern Negev, Israel). Israel Journal of Earth Sciences 20:179–205.
Parnes, A., C. Benjamini, and T. Hirsch. 1985. New aspects of Triassic ammonoid biostratigraphy, paleoenvironments and paleobiography in southern Israel (Sepharadic Province). Journal of Paleontology 59:656–666.
Pettijohn, F. J. 1957. Sedimentary rocks, pp. 400–401. New York: Harper and Row.
Picard, L. 1943. Structure and evolution of Palestine with comparative notes on neighboring countries. Bulletin of the Geology Department, Hebrew University, Jerusalem 4.
Pingree, R. D., P. M. Holligan, and R. N. Head. 1977. Survival of dinoflagellate blooms in the western English Channel. Nature 265:266–269.
Rophe, B., Y. Eyal, and M. Eyal. 1993. The Ramon Laccolith. Israel Journal of Earth Sciences 42:125–131.
Sakal, E., M. Raab, and Z. Reiss. 1966. Upper Eocene in the southeastern Negev. Israel Journal of Earth Sciences 15:42–45.
Sass, E. 1980. Late Cretaceous volcanism in Mount Carmel. Israel Journal of Earth Sciences 29:8–24.
Sass, E., and A. Bein. 1982. The Cretaceous carbonate platform in Israel. Cretaceous Research 3:135–144.
Segev, A. 1984. Lithostratigraphy and paleogeography of the marine Cambrian sequence in southern Israel and southern Jordan. Israel Journal of Earth Sciences 33:26–33.
Segev, A. 1992. Remobilization of uranium and associated metals through kartification processes: A case study from the Timna Formation (Cambrian), southern Israel. Ore Geology Reviews 7:135–148.
Segev, A., and E. Sass. 1989. Copper-enriched syngenetic dolostones as a source for epigenetic copper mineralization in sandstones and shales (Timna, Israel). In Sedimenthosted stratiform copper deposits, ed. R. W. Boyle, A. C. Brown, C. W. Jefferson, E. C. Jowett, and R. V. Kirkham, pp. 647–658. Geological Association of Canada, Special Paper 36.
Seliger, H. H., J. H. Carpenter, M. Loftus, and W. D. McElroy. 1970. Mechanisms for the accumulation of high concentrations of dinoflagellates in a bioluminescent bay. Limnology and Oceanography 15:234–245.
Shimron, A. E., and B. Lang. 1988. New geological data and K-Ar geochronology of the magmatic rocks on the southern flanks of Mt. Hermon. Geological Survey of Israel Report GSI/41/88.
Shlomovitch, N., M. Bar-Matthews, and A. Matthews. 1999. Sedimentary and epigenetic copper mineral assemblages in the Cambrian Timna Formation, southern Israel. Israel Journal of Earth Sciences 48:195–208.
Sigler, R., and V. Wingerden. 1998. Submarine flow and slide deposits in the Kingston Peak Formation, Kingston Range, Mojave Desert, California: Evidence for catastrophic initiation of Noah’s Flood. In Proceedings of the Fourth International Conference on Creationism, ed. R. E. Walsh, pp. 457–501. Pittsburgh, Pennsylvania: Creation Science Fellowship.
Sloss, L. L. 1963. Sequences in the cratonic interior of North America. Geological Society of America Bulletin 72: 93–114.
Sneh, A., Y. Bartov, T. Wiessbrod, and M. Rosensatt. 1998. Geological map of Israel, 1:200,000, 4 sheets. Jersualem: Geological Survey of Israel.
Snelling, A. A. 1994. Can Flood geology explain thick chalk layers? Creation Ex Nihilo Technical Journal 8:11–15.
Snelling, A. A. 1998. Uluru and Kata Tjuta: Testimony to the Flood. Creation 20, no. 2:36–40.
Snelling, A. A. 2007. Geologic evidences for the Genesis Flood. Answers 2, no. 4:81–83.
Snelling, A. A. 2008a. High and dry sea creatures. Answers 3, no. 1:92–95.
Snelling, A. A. 2008b. The world’s a graveyard. Answers 3, no. 2:76–79.
Snelling, A. A. 2008c. Transcontinental rock layers. Answers 3, no. 3:80–83.
Snelling, A. A. 2009a. Beneath the Cross. Answers 4, no. 2:78.
Snelling, A. A. 2009b. Earth’s catastrophic past: Geology, creation and the Flood. Dallas, Texas: Institute for Creation Research.
Snelling, A. A. 2010a. The geology of Israel within the biblical Creation-Flood framework of history: 1. Pre-Flood rocks. Answers Research Journal 3:165–190.
Snelling, A. A. 2010b. Fossilized footprints: A dinosaur dilemma. Answers 5, no. 4:64–67.
Stanley, S. M., J. B. Ries, and L. H. Hardie. 2005. Seawater chemistry, coccolithophore population, and the origin of Cretaceous chalk. Geology 33:593–596.
Starinsky, A., M. Bielski, and G. Steinitz. 1980. Rb-Sr whole rock age of syenitic intrusions (Shen Ramon and Gevnunim) in the Ramon area, southern Israel. Israel Journal of Earth Sciences 29:177–181.
Steinitz, G. 1977. Evaporite-chert associations in Senonian bedded cherts, Israel. Israel Journal of Earth Sciences 26:55–63.
Sumich, J. L. 1976. Biology of marine life, pp. 118, 167. Dubuque, Iowa: William C. Brown.
Sweet, I. P., and I. H. Crick. 1992. Uluru and Kata Tjuta: A geological history. Canberra, Australia: Australian Geological Survey Organisation.
Vermeesch, P., D. Avigad, and M. O. McWilliams. 2009. 500 m.y. of thermal history elucidated by multi-method detrital thermochronology of north Gondwana Cambrian sandstone (Eilat area, Israel). Geological Society of America Bulletin 121:1204–1216.
Visher, G. S. 1990. Exploration stratigraphy, 2nd ed. Tusa, Oklahoma: Penn Well Publishing Co.
Weissbrod, T. 1969. The Paleozoic of Israel and adjacent countries: The Paleozoic stratigraphy of southern Israel. Geological Survey of Israel Bulletin 47.
Wells, A. T., D. J. Forman, L. C. Ranford, and P. J. Cook. 1970. Geology of the Amadeus Basin, Central Australia. Bulletin 100, Bureau of Mineral Resources, Australia.
Whitmore, J. H. 2006a. The Green River Formation: A large post-Flood lake system. Journal of Creation 20, no. 1:55–63.
Whitmore, J. H. 2006b. The geologic setting of the Green River Formation. Journal of Creation 20, no. 1:72–78.
Whitmore, J. H. 2006c. Difficulties with a Flood model for the Green River Formation. Journal of Creation 20. no. 1: 81–85.
Whitmore, J. H., and P. Garner. 2008. Using suites of criteria to recognize pre-Flood, Flood and post-Flood strata in the rock record with application to Wyoming (USA). In Proceedings of the Sixth International Conference on Creationism, ed. A. A. Snelling, pp. 425–448. Pittsburgh, Pennsylvania: Creation Science Fellowship, and Dallas, Texas: Institute for Creation Research.
Wilson, W. B., and A. Collier. 1955. Preliminary notes on the culturing of Gymnodinium brevis Davis. Science 121: 394–395.
Wingerden, V. 2003. Initial Flood deposits of the western North American Cordillera, California, Utah and Idaho. In Proceedings of the Fifth International Conference on Creationism, ed. R. L. Ivey, Jr., pp. 349–358. Pittsburgh, Pennsylvania: Creation Science Fellowship.